ABSTRACT
The fundamental assumptions of conventional solar flare and coronal mass ejection (CME) theory are re-examined. In particular, the common theoretical assumption that magnetic energy that drives flares and CMEs can be stored in situ in the corona with sufficient energy density is found wanting. In addition, the observational constraint that flares and CMEs produce non-thermal electrons with fluxes of order 1034–1036 electrons s−1, with energies of order 10–20 keV, must also be explained. This constraint when imposed on the "standard model" for flares and CMEs is found to miss the mark by many orders of magnitude. We suggest, in conclusion, there are really only two possible ways to explain the requirements of observations and theory: flares and CMEs are caused by mass-loaded prominences or driven directly by emerging magnetized flux.
Export citation and abstract BibTeX RIS
1. INTRODUCTION
Large solar flares and coronal mass ejections (CMEs) are characterized by the release of approximately 1032 erg in approximately 10–1000 s (a giant flare with a yield of 1034 erg has been reported (Kane et al. 1995), although this may be a one-off case). The release of this energy within the exponential atmosphere of the Sun manifests itself in a variety of forms, including particle acceleration and large-scale bulk mass motions. The canonical explanation for both flare and CME phenomena4 is that magnetic free energy is stored in situ within the solar corona and rapidly dissipated by some mechanism, usually assumed to be magnetic reconnection although with little, if any, definitive proof. Our objective here is to re-examine the in situ assumption since, as will become apparent, there are a number of consequences that follow from it that are physically objectionable. In addition, we examine whether the canonical model of flares and CMEs, the so-called "standard model," can accelerate by reconnection the requisite electron fluxes known to be necessary to explain the observed hard X-rays observed during flares and CMEs. Our result shows that the standard model in its present form cannot meet this requirement.
2. THEORY
The magnetic energy required for liberation during a typical large flare is estimated as follows:

where B = Bo + B⊥, Bo is the vacuum magnetic field, B⊥ is due to currents mainly parallel5 to Bo (Bo · B⊥ ∼ 0), L is the size of the magnetic active region, and = B⊥/Bo ≪ 1. As it is difficult to estimate the total energy available to power a flare or a CME, since we have no real estimate of how the magnetic field varies with height if it is non-potential, we will assume that the magnetic free energy is constant throughout the entire volume L3. Thus, the estimates we give here are at best upper limits for the flare or CME free energy. Taking L ∼ 105 km, Bo ∼ 102 G, and
∼ 0.2, we obtain
erg and
erg, which barely approaches the observed energy release from a large flare.6 The available energy can also be estimated from the observed magnetic field at the photospheric surface through the relation (Chandrasekar 1961; Low 1982)

where the z = 0 plane represents the photosphere. Although observations of the photospheric magnetic field lead to a figure of ∼1032 erg, the uncertainty in the measurements of is of order
. Taking a larger value for L puts considerable strain on the mechanisms, such as magnetic reconnection and annihilation, that would explain the release of this energy in 10–1000 s. The fact is that this time is only 10–100τA, where τA = L/VA is itself unexplained (Alfvén velocity VA ∼ 1000–2000 km s−1) by any acceptable magnetic reconnection process. Likewise choosing a larger value for B would restrict L to a smaller value since larger magnetic fields would be associated with smaller regions. We conclude that the available free energy in the corona is embarrassingly marginal, especially when the efficiency of the processes that convert magnetic energy to X-ray radiation have to be taken into account and given that a sizable fraction of the energy output of a solar flare and CMEs can be in soft and hard X-rays, as well as, bulk mass motions.
2.1. Force-free Currents and Magnetic Energy Density Constraints
Directly related to the total energy required to explain a CME or a flare is the magnetic energy density , which is distributed throughout the volume L3. Often forgotten, however, is the requirement that the magnetic energy density itself must have constraints imposed on it. High magnetic energy densities are required to drive any magnetic dissipation mechanism if that dissipation mechanism is to release the flare or CME energies on the timescales observed. This is not a trivial constraint because high magnetic energy densities imply that high magnetic pressures must be embedded somewhere in a low-pressure exponential atmosphere and contained by some force, as required by the virial theorem (Chandrasekar 1961). The canonical view is that because currents are force free there are no issues with containment. This argument is highly dubious, at best, since the solar atmosphere is not a quiescent volume of space, but a region being constantly jostled by photospheric motions that are not all small amplitudes. Assuming that magnetic structures in the solar atmosphere are force free, J × B = 0, is thus more a mathematical than a physical argument.
Consider the force-free assumption J × B = 0. What is usually done is to simply solve the equation which the force-free assumption implies, ∇ × B = αB, with some mathematically convenient boundary conditions on B. While mathematically acceptable, it is not physically acceptable. One reason is immediately obvious. The solution to ∇ × B = αB in no way constrains the value of α and thus J, permitting any value for B desired. From the global virial theorem, which simply states that no finite magnetic field-plasma configuration can be in stress balance by itself without the aid of external stresses (Chandrasekar 1961), we know we must identify what these external stresses are and then impose them so as to provide realistic meaning to solutions of ∇ × B = αB. To determine what these external stresses are and to determine α, we use the MHD momentum equation

to determine J⊥

hence

Since ∇ · J = 0 in MHD, it follows that

and this requirement, when applied to Equation (5), yields

or

where s is measured along a field line. As the magnetic systems we are considering are not usually closed, and field lines can both enter and leave the volume of interest, it follows that, if there are no currents in the region outside the volume of interest we must have

where the integral is taken from the point where the field line enters the plasma volume of interest and to where it leaves it. Further, if Equation (9) is satisfied, a unique α can always be constructed from Equation (8). This condition is therefore necessary and sufficient (Taylor 1963). On the other hand, if there are currents outside of the domain of interest there is no guarantee of a unique α, which most solutions to ∇ × B = αB implicitly assume. This latter point can dramatically complicate force field models because there is a high likelihood that there are external currents, particularly at the photosphere originating in the convection zone. In addition to this result, Equation (6) identifies the external stresses required by the virial theorem as any of the terms contained in Equation (4). Thus the procedure to determine α, and thus the magnitude of the magnetic field energy provided by the field-aligned current density, is to integrate Equation (8) subject to the constraint (Equation (9)). Only then can we be certain that a force field solution has sufficient free magnetic energy stored in situ to explain a flare or a CME. In addition, the solution, assuming it is analytic, can be tested for stability. Without stability, field-aligned current systems will not exist for very long in the solar atmosphere. With the notable exceptions of Low & Murdin (2000), Gibson & Low (2000), and Low (2001) this conclusion is universally ignored, resulting in the erroneous conclusion that large magnetic energy densities can be stably stored in a low-pressure exponential atmosphere without a non-magnetic force containing the high energy densities.
2.2. Magnetic Storage and Tangential Discontinuities
Dissipation is usually viewed as occurring through the formation of current sheets or tangential discontinuities of the magnetic fields, which are regions where the normal component of the magnetic field vanishes (Parker 1972). Physically, this has significant import because such regions cannot couple to Alfvén waves since it is through Alfvén waves that most MHD systems shed Maxwell stresses. While we have no intention of discussing Parker's hypothesis in any detail, i.e., that current sheets or tangential discontinuities of the magnetic field can occur in the absence of genuine X-points, it is the case that this hypothesis is often used to argue that if tangential discontinuities are ubiquitous in solar magnetic systems (as is implied), then it would be impossible to build up and store sufficient magnetic energy to explain a flare or a CME. If this argument were true, then our work here would be done. However, we do not share the view that tangential discontinuities are as ubiquitous as has been argued, since the kind of velocity fields needed to cause tangential discontinuities must also be ubiquitous throughout a magnetic configuration, and they have not been definitively shown to exist observationally or theoretically (care must be exercised when results from numerical models are invoked, since such simulations invariably impose boundary conditions that by design result in stagnation flows and thus reconnection). As we will show, there are far more compelling reasons against in situ magnetic energy storage.
2.3. Magnetic Storage and Types of Magnetic Configurations
It is often stated in the literature that quasi-equilibrium configurations exist in the solar atmosphere in which magnetic energy can be slowly built up and stored. There are three types of clearly definable magnetic configurations in the solar atmosphere: open fields, loops, and prominences. Open magnetic field configurations, if stressed as is usual by photospheric motions, simply shed those stresses by Alfvén waves propagating out into the open solar heliosphere. Loops and prominences represent a more complex situation.
First consider the loop. Assume it is initially in pressure balance with its surrounding. If the magnetic field is a potential field, the loop will remain at its equilibrium height unless the internal energy density within the loop changes. If the loop cools it will contract, and if it heats up it will expand, in each case again reaching quasi-equilibrium. To increase the magnetic energy density within the loop stresses must be applied at the loop's feet since it is only there, if you ignore mass loading, that the energy density in all its possible forms is great enough to cause any stressing of the loop's magnetic field. Assume photospheric motions cause one foot, or both feet, to shear in such a way as to cause a large amplitude long wavelength Alfvén wave to form, thereby setting up a field-aligned current system (Spicer 1983). As the shearing increases there will be a steady buildup of the poloidal component of the magnetic field. It is not hard to see that the gradient in the poloidal component of the magnetic field is pointed at the apex of the loop in such a direction as to cause the loop to expand outward and thus lower the energy density of the poloidal component of the magnetic field. This represents an additional problem for flare models that claim that magnetic energy is slowly stored in situ in the corona, because even though the total magnetic energy is increasing, the magnetic energy density needed to drive the flare or CME on the needed timescales is decreasing, not increasing as is required. The only means by which one can avoid this problem is to mass load the magnetic field configuration, that is, use the gravitational force to keep the magnetic field from expanding, such as appears to occur with prominences.
Can mass loading solve the dilemmas outlined above? The gravitational energy MgH is the energy available when a mass M falls through a height H. From Equation (4), we can see that gravity can act as a current source. What is not appreciated is that a gravitationally driven transverse current must close within the magnetic configuration because of the ∇ · J = 0 requirement. Thus, a current system is set up with current density components both parallel and perpendicular to the magnetic field. Such a current system can cause magnetic energy to grow for a limited amount of time at the expense of gravitational potential energy. The maximum time the current system can grow is controlled by at least two factors: the stability of the entire system to the growth of the magnetic field due to the growth of the net current and the resistive time. The resistive time is the least understood of these two effects because it is controlled by the geometry of the magnetic field/current system and the locations and types of dissipative effects to be found in the structure. To see this assume a straight and uniform magnetic field in the x-direction ending on two dissipative end plates at x = ±ℓ. If we now apply a gravitation force, Mg in the z-direction at x = 0, a current will flow in the y-direction at x = 0. This will cause currents to flow in anti-parallel directions on both sides of the current source at x = 0 toward the end plates. The net current in the system flowing toward the plates is initially zero by Lenz's law. However, as time progresses the net current will grow as Inet = Io(1 − e−t/τ), where Io = V/R, with V being the applied voltage produced by the force of gravity perpendicular to B, R the integrated resistance of the global circuit, and τ the resistive time constant. τ is determined by the total impedance of the circuit and will allow a net current to grow given by Io = V/R. This current represents inductive energy storage, , where L is the inductance, which is of order ℓ/c. However, since V is controlled by gravity the total energy inductively stored in the circuit cannot exceed the gravitational energy MgH. The conclusion one can draw from this is that unless the gravitational energy MgH is large enough to provide all the energy released during a flare or a CME, with some to spare due to inefficiencies, mass loading cannot be the principal source of energy released during a flare. In fact, the magnetic field produced by the net current will very likely destabilize the magnetic structure well before
becomes comparable to MgH. If this occurs a catastrophic instability such as a magnetized Rayleigh–Taylor instability will dump some of the mass or some form of internal reconnection due to tangential discontinuities will allow the magnetic stresses, built up due to mass loading, to be relaxed and this relaxation will manifest itself, in part, as a rising of the magnetic field (the speed of the rising will depend on the nature of the relaxation process; slow relaxation means slow rise, and so on) that previously supported the mass M.
The conclusion that the available energy due to mass loading is really all the energy available for release during a CME or similar event, and the fact that MgH is really not that well known observationally argues in favor of a CME possibly being caused by mass loading because of many observed features associated with the CME's behavior during the eruption process. A simple physical argument (Spicer et al. 2006) that supports the idea that twisted/sheared magnetic fields exist in prominences, such as would be caused by mass loading, may be responsible for the eruption of CMEs is as follows: it is simple to show, assuming the majority of magnetic flux, ϕ, within a prominence or emerging flux is conserved during an eruption (local reconnection needed to allow for relaxation of MHD stresses will not appreciably change our argument) that the total magnetic energy is just
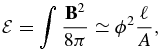
so that the magnetic energy varies with time as
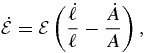
where ℓ is the length the magnetic structure and A is the area enclosing the magnetic field structure. This expression shows that magnetic energy is reduced in time primarily by the expansion of magnetic flux "rope" often seen at the beginning of a CME (Harrison 1986, 1995; Harrison et al. 1990; Savani et al. 2009). As the rope unwinds and rotates, the area increases rapidly with time. This effect is exactly what occurs when a stabilized "z-pinch" goes kink unstable.7 This picture is consistent with the fact that CMEs appear to arise from small volumes, eventually becoming 45 degrees across. This picture is also consistent with the homologous flare, if only a fraction of the magnetic field expands outward, as relaxation occurs through a simple localized reconnection event, such as a resistive kink (Spicer 1982), thereby leaving the initial magnetic field configuration pretty much intact.
2.4. Homologous Flares and CMEs
The term homologous itself suggests what these events represent: homology (Merriam-Webster says: a similarity often attributable to common origin). These events repeat themselves in such a way that it appears the global magnetic configuration changes very little. The lack of major magnetic field configuration restructuring suggests two important points: reconnection is not playing the fundamental role often attributed to it and little internal magnetic energy has been expended to cause the event. Neither of these is consistent with a model that uses force-free fields as a magnetic storage medium.
2.5. Energy Constraints and Observational Constraints Imposed by the Hard X-Ray Hypothesis
One additional potential problem with the present explanation for flares is that a substantial fraction of the flare energy is observed to be in the form of hard X-rays (>10 keV) with a flux significantly large that if they are interpreted as due to non-thermal bremsstrahlung, the energy in the electrons producing the X-rays would be as much as, or more than, 50% of the total flare budget. Recent YOHKOH and RHESSI data continue to support this interpretation (Lin et al. 2003; Sui et al. 2005; Hudson et al. 2006). If the non-thermal interpretation of the flare hard X-rays is indeed correct, then we have a paradox. The paradox is that placing 50% or more of the flare energy directly into electrons implies an extremely efficient acceleration mechanism. However, a mechanism with such an efficiency is highly unlikely since there are many other modes into which the flare energy can go other than just electrons. An efficiency of acceleration like 5%–10% is more realistic, and even that may be too high, given evidence from both laboratory and other space physics phenomena like magnetospheric sub-storms. The solution to this paradox is to argue either: (1) the hard X-rays are really formed by the more efficient mechanism of thermal bremsstrahlung (Brown et al. 1979) (the principal argument against a thermal bremsstrahlung model is primarily the short timescales characterizing the fine structure in the hard X-ray bursts and the accompanying similar fine structure in the clearly non-thermal radio bursts) or (2) that the total flare energy is much larger than previously appreciated. If explanation (2) is correct and we assume a 5%–10% efficiency for non-thermal electron acceleration, it follows that the total energy involved in a flare must be roughly 10–20 times larger than is usually assumed. Could this be the case? As noted above, there is a serious problem storing stably sufficient magnetic energy in situ to explain a flare or a CME, so increasing the requirement by a factor of 10–20 or more would only exacerbate the problem. Can any present model for flares and CMEs even meet the electron flux requirements imposed by hard X-ray bursts? Let us examine the canonical model of solar flares and CMEs, the standard model (Hirayama 1974; Kopp & Pneuman 1976), to see if such a model can generate the requisite non-thermal electrons. This model requires huge volumes for it to work energetically and at relatively high magnitudes for the magnetic fields and for these reasons is already suspect as a flare or a CME model, but if it cannot produce the observational requirements of the non-thermal hard X-rays it will need serious revision or abandonment. In a nutshell, hard X-ray events observed during both CMEs and flares typically require 1034–1036 electrons s−1 with energies between 10 and 20 keV, or roughly 1.6 × 1026–3.2 × 1028 erg s−1 (Brown et al. 1979; Sui et al. 2005) if the hard X-rays are non-thermal. This is a solid observational test any CME or flare model must pass.
2.5.1. The Failure of the Standard Model to Produce the Needed Non-thermal Electrons Required of Hard X-Ray Observations
In all current sheet reconnection models of CMEs and flares (the standard model being the most widely accepted model), which assume anti-parallel magnetic fields, the ratio of the current layer thickness, a, to the diffusion region length, L, satisfies the condition a/L ≪ 1 (Cravens 1997). This condition imposes the constraint that the inflow speed of the plasma into the reconnection region Uin = aVAin/L ≈ rciVAin/L for a collisionless plasma, where VAin is the inflow Alfvén speed and rci is the ion gyroradius. Physically, this implies that a large fraction of the inflow magnetic energy, , is converted to bulk outflow kinetic energy, leaving little inflow magnetic energy to either heat or accelerate electrons and ions within the diffusion region where they can become unmagnetized and thus easily accelerated,8 where Bin is the magnitude of the inflow magnetic field. The rate at which electrodynamic energy is transported into the diffusion region is just the Poynting flux so that the power flowing into the current sheet is

where Lℓ is the area through which the energy flux flows and ℓ being the length of the physical dimension orthogonal to the reconnection plane. Here ℓ will be assumed to be of the order of a solar radius. On the other hand, the length of the diffusion region, L, is more difficult to estimate unless a reconnection model is assumed, which we take to be a Petschek–Sweet model assuming collisionless reconnection (this assumption will not radically alter our final conclusion). In collisionless reconnection, Cowley (1985) estimates the minimum value for L is of the order Lmin ≈ 10rci–50rci, thus
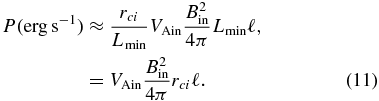
This result9 represents the maximum power available to both heat and/or accelerated particles. If we use ℓ = 7 × 1010 ℓ☉, Bin = 102B2, and VAin = 108 V8Ain while leaving rci as is we find

Equation (12) demonstrates that unless extreme values are assumed it is physically impossible to accelerate adequate electrons to explain the non-thermal hard X-ray bursts. To reinforce this result we assume plasma quasi-neutrality, ne ≈ ni, and compute the number of electrons s−1 flowing into the current sheet and compare the result with the electron s−1 needed by the hard X-ray hypothesis. The number of electrons s−1 flowing into the current sheet is just


It should be clear from Equation (14) that even if all of the electrons s−1 flowing into the sheet were to be accelerated, the result would still fall 4–6 orders of magnitude short of what is needed.
It should now be clear that the standard model is well short of the mark as far as meeting the observational constraint that 1034–1036 electrons s−1 be accelerated directly by a reconnecting current sheet. Given this clear problem with the standard model, is there a method for saving reconnection as a direct particle acceleration mechanism for CMEs and flares? Simply positing roughly 106 or more reconnection sites could save reconnection but this is highly doubtful since the magnetic configurations needed to produce such large numbers of X-points are very unlikely to be found in the solar atmosphere.10 Research during the Skylab workshop series (Sturrock 1980) and more recently by Emslie et al. (2005) demonstrates that the bulk of the flare and CME energy appears to be in mass motions, not in non-thermal particles. These results, combined with ours, strongly suggest that mass flows set in motion by the reconnection relaxation of mass-loaded stressed magnetic fields or emerging directly from the photosphere is the particle acceleration driver (Spicer et al. 2006) and not directly by reconnection electric fields, as is usually assumed in the standard model. Spicer et al. (2006) suggest three alternative methods of accelerating the non-thermal electrons: betatron acceleration (Glasstone & Lovberg 1960), lower hybrid wave acceleration (Lampe & Papadopoulos 1977; Spicer et al. 1981, 1990; McClements et al. 1990, 1993), and kinetic Alfvén waves (Hasegawa 1976; Goertz & Boswell 1979; Lysak 1990; Lysak & Song 2000; Spicer 1982; Hudson & Fletcher 2007; Bingham et al. 2009), the latter two being driven by bulk flows that very likely give rise to magnetized pistons (Spicer et al. 1990). In the original Kopp & Pneuman (1976) paper it was suggested that during the dipolarization process, which results in the formation of post-flare loops, betatron acceleration could be an explanation for the observed hot post-flare loops. Independent work by Brown & Hoyng (1975) showed that betatron acceleration could explain hard X-ray bursts and thus is consistent with the standard model (Hirayama 1974; Kopp & Pneuman 1976) picture. In addition, as we have shown here, the acceleration of electrons cannot be due to electric fields generated by reconnection, adding further support to the betatron hypothesis of Kopp & Pneuman (1976) and demonstrated as a workable acceleration mechanism by Brown & Hoyng (1975).
3. CONCLUSIONS
So what are the possible solutions to the dilemma of what causes flares and CMEs? In our opinion there are only two clear options for which all the problems discussed here disappear. The first solution requires the magnetic energy, in the form of strongly sheared magnetic fields that drive the flare, be stored sub-photospherically prior to the flare, and it is the emergence of this sub-photospherically stored magnetic shear energy into the photosphere and above, and its rapid conversion to other forms, that is the actual flare. Note this is not the equivalent of the emerging flux model of a solar flare, where the emerging flux acts as a trigger that permits the release of in situ coronal stored magnetic energy to cause the flare or CME. On the contrary, we are arguing that the rapidly emerging flux is the flare. With this scenario, the electron acceleration efficiency problem and the stable storage of free magnetic energy problem disappear simultaneously. The second option is the classic mass-loaded prominence model of Kippenhahn & Schlüter (1972) which morphed into the standard model (Hirayama 1974; Kopp & Pneuman 1976) but with the major modification that the observed hard X-rays are not produced by the reconnecting sheet. This option can only be made viable if it can be observationally demonstrated that the mass height relationship of order MH ≈ 3.6 × 1027 g cm is possible. Thus the focus of future research should be directed toward a systematic study of magnetic flux emergence and the better observational measures of the mass stored in prominence because both contain the keys to the explanation of large solar flares and CMEs.
Footnotes
- 4
While flares and CMEs are often treated as separate phenomena theoretically most models purported to explain each in fact are very similar and therefore essentially "dual" use models. Thus many of our arguments about one are true about the other.
- 5
This is assuming that the magnetic fields are essentially force free. If a non-magnetic force contains the magnetized plasma such as due to mass loading
⩾ 1 is possible.
- 6
We have selected a value of 0.2 to reflect the fact that homologous flares and CMEs show little, if any, change to the magnetic field structure, see Section 2.4.
- 7
Neither mass-loaded filaments nor emerging flux are subject to the Kruskal–Shafranov limit and therefore the number of twists in the magnetic field can greatly exceed the usual number of ∼1.5 or so.
- 8
- 9
These results are based on mass and energy conservation and will not be altered by kinetic arguments.
- 10