ABSTRACT
The influence of nonthermal shielding on the optically allowed and forbidden anti-screening channels for ion–ion collisional excitations is investigated in astrophysical Lorentzian plasmas. The semiclassical trajectory method and effective interaction Hamiltonian are employed to obtain the transition amplitudes, differential cross-sections, and momentum transfer-dependent effective projectile charges for the optically allowed and forbidden excitation channels as functions of the impact parameter, collision energy, Debye radius, and spectral index of nonthermal astrophysical plasmas. It is found that the nonthermal effect suppresses the ion–ion collisional excitation probability in astrophysical Lorentzian plasmas. Additionally, the influence of nonthermal shielding on the optically allowed transition is found to be more significant than that on the optically forbidden transition. The variations of the nonthermal shielding effects on the optically allowed and forbidden anti-screening channels in astrophysical nonthermal plasmas are also discussed.
Export citation and abstract BibTeX RIS
1. INTRODUCTION
The atomic collision and radiation processes in astrophysical thermal or nonthermal plasmas have received considerable attentions since these atomic processes have been widely used as plasma diagnostic tools in astrophysical plasmas (Cravens et al. 1975; Cravens & Dalgarno 1978; Dalgarno & Layzer 1987; Tawara et al. 1990; Jung 1992, 1993; Shevelko & Vainshtein 1993; Ferland et al. 1997; Shevelko 1997; Shevelko & Tawara 1998; Cox 2000; Flower 2000; Hutchinson 2002; Rau 2002; Gould 2006; Yoon et al. 2008; Tolstikhina et al. 2014). Recently, the atomic collisional excitation process has been extensively investigated in order to obtain the information on the plasma parameters via the line-emission spectra from excited states (Fujimoto 2004; Osterbrock & Ferland 2006; Shevelko & Tawara 2012). It also has been shown that the ion–ion collisional excitations have provided various line spectra from the double-excitation states (McGuire 1997). Additionally, it has been shown that the ion–ion collisional excitation process would have two different excitation channels such as the screening and anti-screening excitation mechanisms (Eicher & Meyerhof 1995). Further, it has been shown that the anti-screening excitation channel (i.e., projectile inelastic and target inelastic) is quite different from the screening excitation channel (i.e., projectile elastic and target inelastic) owing to the effective interaction between the bound electron in the projectile ion and the target nucleus (Yoon & Jung 1997, 1999). Generally, the atomic collision processes in weakly coupled thermal plasmas have been extensively explored by using the effective Debye–Hückel screening model since the screened interaction energy between charged particles is usually smaller than the average kinetic energy of a plasma particle (Jung 1993; Baumjohann & Treumann 1996; Ramazanov & Dzhumagulova 2002). However, it has been shown that the particle acceleration mechanism owing to the coupling of the Maxwellian plasma with the external field readily generates super-thermal particles that are quite different from the thermal equilibrium distributions in nonthermal astrophysical plasmas such as magneto-tail near astrophysical plasma shock waves, solar atmosphere, and solar-wind plasmas (Hasegawa et al. 1985; Hasegawa & Sato 1989; Scudder 1992; Mendis & Rosenberg 1994). Additionally, in various astrophysical plasmas it has been shown that the plasma electrons that escaped from the thermal Maxwellian distribution would be effectively represented quite well by the Lorentzian form of the distribution function since the interaction potential in nonthermal astrophysical plasmas would not be appropriately expressed by the standard form of the screened Debye–Hückel potential owing to the influence of collective interactions of nonthermal plasma particles (Hasegawa & Sato 1989; Rubab & Murtaza 2006; Jung 2009; Ki & Jung 2012, 2013). Hence, we can expect that the ion–ion anti-screening collisional excitation process in astrophysical nonthermal Lorentzian plasmas would be quite different from those in thermal Maxwellian plasmas owing to the nonthermal shielding character and unsymmetrical portion of high-energy tails of the astrophysical Lorentzian plasma. However, the nonthermal shielding effect on the ion–ion anti-screening collisional excitation has not been investigated as of yet. Thus, in this paper we investigate the influence of nonthermal shielding on both the optically allowed and forbidden anti-screening channels for ion–ion collisional excitations in astrophysical Lorentzian plasmas. The semiclassical trajectory method is employed to obtain the Hamiltonian matrix elements and transition amplitudes for the ion–ion anti-screening collisional excitations. The screened ion–ion interaction Hamiltonian in astrophysical Lorentzian plasmas is obtained by taking into account the physical behavior of the bound electrons in the projectile ion and also in the target ion including the effective nonthermal shielding distance. The momentum transfer-dependent effective projectile charges and transition probabilities for the optically allowed and forbidden ion–ion collisional excitations are also obtained as functions of the impact parameter, collision energy, Debye radius, and spectral index of astrophysical Lorentzian plasmas.
This paper is composed as follows. In Section 2, we discuss the hydrogen-like ion–ion interaction Hamiltonian in astrophysical nonthermal Lorentzian plasmas. In Section 3, we derive the transition amplitudes for the ion–ion collisional excitations in nonthermal Lorentzian plasmas. In Section 4, we obtain the transition probabilities as well as differential cross sections for the optically allowed and forbidden anti-screening excitations in astrophysical Lorentzian plasmas. In Section 5, the nonthermal shielding effects on the anti-screening optically allowed and forbidden ion–ion collisional excitations are investigated in astrophysical nonthermal Lorentzian plasmas. Finally, the conclusions are given in Section 6.
2. INTERACTION HAMILTONIAN IN LORENTZIAN DISTRIBUTION
In numerous astrophysical and space plasmas such as supernova remnants and warm ionized medium, it has been known that the external interaction in thermal plasmas readily produces the high-energy plasma electrons that escaped from the standard Maxwellian distribution. Additionally, it is found that the unsymmetrical nonthermal distribution function owing to the high-energy portion of super-thermal plasma electrons departed from the thermal Maxwellian distribution and also owing to the entropy generalization mechanism would be represented effectively by the power-law form of the Lorentzian distribution function (Hasegawa et al. 1985; Leubner 2004; Ki & Jung 2012, 2013) fL(v; κ) given by

where m is the mass of the electron, κ(> 3/2) is the spectral index of the astrophysical nonthermal Lorentzian plasma, Eκ[ ≡ (κ − 3/2)EM/κ] is the characteristic energy of the plasma electron in Lorentzian plasmas, EM ≡ kBT, kB is the Boltzmann constant, T is the plasma temperature, and Γ(x) represents the gamma function with the argument x. It is interesting to note that the astrophysical nonthermal Lorentzian distribution yields the thermal Maxwellian distribution form for all velocities when the spectral index κ of the plasma goes to infinity, i.e., in the absence of the external interaction (Hasegawa et al. 1985; Hasegawa & Sato 1989): fL(v; κ → ∞)∝exp (− mv2/2Eκ → ∞), where Eκ → ∞ → kBT(= EM) corresponds to the Maxwellian characteristic energy in thermal plasmas. From Equation (1), the astrophysical Lorentzian distribution function at high energies would be a simple power-law form: fL(v; κ)∝(mv2/2κEκ)−(κ + 1). Additionally, the effective nonthermal screening length λκ, i.e., the critical correlation distance, in astrophysical nonthermal Lorentzian plasmas has been obtained by the following relation (Rubab & Murtaza 2006): λκ ≡ μκλD, where μκ{ ≡ [(κ − 3/2)/(κ − 1/2)]1/2} is known as the characteristic nonthermal factor that represents the measure of the fraction of the suprathermal population in the astrophysical Lorenzian distribution function and λD stands for the standard Debye length in Maxwellian plasmas. It is shown that the coupling with the external radiation field in nonthermal Lorentzian plasmas causes the additional diffusion correction (Hasegawa & Sato 1989) along with the conventional Coulomb diffusion effect. It is also found that the Coulomb mean free path in astrophysical nonthermal Lorentzian plasmas is quite large, thus the plasma particles would not have enough time to reach the equilibrium states. Using the nonthermal screening length with the Lorenzian distribution, the screened interaction between the one-electron target ion and the one-electron projectile ion in astrophysical nonthermal Lorentzian plasmas is obtained by the following form of the effective Hamiltonian Hint(rT, rP, R, κ):
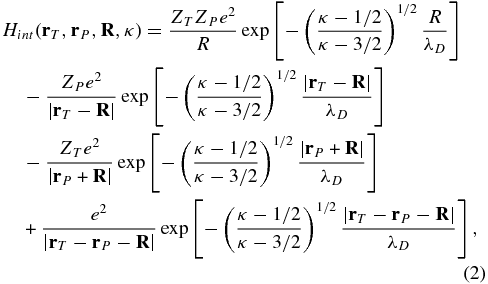
where rT is the coordinate of the target electron with respect to the target nucleus ZTe, rP is the coordinate of the projectile electron with respect to the projectile nucleus ZPe, e is the elementary charge of the electron, and R is the position of the projectile nucleus with respect to the target nucleus. In the semiclassical trajectory analysis, the position of the projectile ion would be represented by a function of time R(t).
In the first-order semiclassical approximation (McGuire 1997), the hydrogen-like ion–ion collision cross section for the excitation from an unperturbed initial state of the collision system to an excited final state
is represented by

where and
are, respectively, the initial and final wave functions for the bound electron in the target system,
and
are the initial and final wave functions for the bound electron in the projectile system, b is the impact parameter, and the transition amplitudes
for the excitations iP → fP and iT → fT are given by the following integral representation of the Hamiltonian matrix elements:

where ωf, i[ = (Ef − Ei)/ℏ] is the transition frequency, and
are the total energy eigenvalues of the final state |.fT, fP〉. and initial state |.iT, iP〉. of the collision system, and ℏ is the rationalized Planck constant.
3. TRANSITION AMPLITUDES FOR ANTI-SCREENING CHANNELS
For the ion–ion collisional double excitations, we can now consider the two cases of the final state such as |.fT ≠ iT, fP = iP〉. (target inelastic and projectile elastic) and |.fT ≠ iT, fP ≠ iP〉. (target inelastic and projectile inelastic). Here the final states represented by |.fT ≠ iT, fP = iP〉. and |.fT ≠ iT, fP ≠ iP〉. are known as the screening and anti-screening excitation channels, respectively, since the excitation cross sections for the target-inelastic collisions are always greater than those for the target-elastic collisions (Eicher & Meyerhof 1995). In these anti-screening excitation channels in astrophysical nonthermal plasmas, the first screened interaction term, (ZTZPe2/R)exp [ − (κ − 1/2)1/2R/(κ − 3/2)1/2λD], and the third screened interaction term, (− ZTe2/|rP + R|)exp [ − (κ − 1/2)1/2|rP + R|/(κ − 3/2)1/2λD], in Equation (4) do not have any contributions to the transition amplitudes owing to the orthogonality of the initial and final target states, i.e.,
. After some mathematical manipulations, the transition amplitudes
for the anti-screening ion–ion collisional excitations are represented by
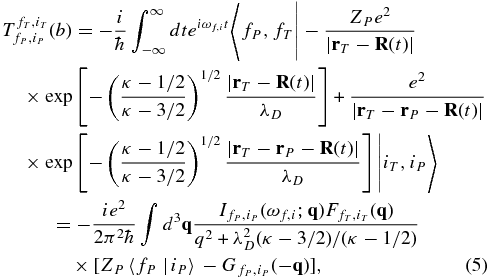
where the integral function represents the dynamic structure factor:

stands for the target form factor
,
represents the projectile form factor
, and R(t) is the time-dependent inter-nucleus position vector. For conventional heavy ion collisions, the projectile path would be represented by the straight line such as R(t) = b + vt with b · v = 0, where v is the collision velocity, since it has been known that the straight-trajectory analysis (Bethe & Jackiw 1986) is quite reliable for
, where μ is the reduced mass of the collision system and Ry(= me4/2ℏ2 ≈ 13.6 eV) is the Rydberg constant. Hence, if we set the momentum transfer as q = q∥v/v + q⊥, where q∥ and q⊥ are, respectively, the parallel and perpendicular components of the momentum transfer with respect to the velocity direction, the transition amplitudes
for the anti-screening ion–ion collisional excitation would be represented by

where is the momentum transfer-dependent projectile charge number in nonthermal Lorentzian plasmas:
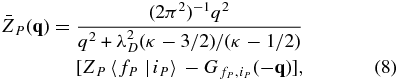
and the dynamic structure factor (Yoon & Jung 1997) can also be represented by the following form:
.
4. TRANSITION CROSS SECTIONS FOR ANTI-SCREENING CHANNELS
From Equation (2), the differential excitation cross section is given by

where is the scaled impact parameter,
is the Bohr radius of the hydrogenic ion with nuclear charge ZTe, and
is the transition probability for the ion–ion collisional excitation. Using the hydrogenic wave functions (Bethe & Salpeter 1957), the projectile form factor
and target form factor
for the optically forbidden (1s → 2s) and allowed (1s → 2p) transitions in the ion–ion collisional excitations are found to be
,
,
, and
, where the static structure functions SS(q, a) and SP(q, a) are given by


and the final excited substate becomes the state 2p(mf = 0), i.e., 2p0 or 2pz state, mf is the magnetic quantum number of the final state, and is the Bohr radius of the hydrogenic ion with nuclear charge ZPe. For the sake of simplicity, we set ZP = ZT ≡ Z, and then the momentum transfer-dependent effective projectile charge number (McGuire 1997)
for the optically forbidden (1s → 2s) and allowed (1s → 2p) transitions in astrophysical nonthermal Lorentzian plasmas are, respectively, found to be


where Q(≡ qaZ) is the scaled momentum transfer, aZ(= a0/Z) is the Bohr radius of the hydrogenic ion with nuclear charge Ze, and aD(≡ aZ/λD) is the scaled reciprocal Debye length. In order to specifically investigate the nonthermal shielding effects on the ion–ion collisional excitations in astrophysical nonthermal Lorentzian plasmas, we consider two cases of the excitation transitions such as the optically forbidden–forbidden transitions and allowed–allowed transitions
since the excited 2s state only decays into the ground 1s state by the two-photon process (Weinberg 2013). Since it has been shown that the optically allowed transitions dominate the total excitation cross section at high energies (Joachain 1983), we consider the optically allowed–allowed transition in order to investigate the influence of nonthermal shielding on the ion–ion collisional excitation process. After some mathematical manipulations in the cylindrical coordinate for the azimuthal angle between q⊥ and b, the scaled differential cross sections
in units of
for the optically forbidden–forbidden
and allowed–allowed
transitions in astrophysical nonthermal Lorentzian plasmas owing to the ion–ion collisions are then, respectively, obtained in the following forms:
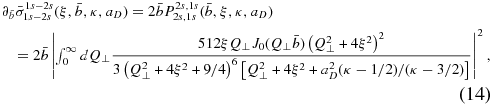
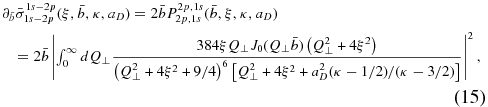
where ξ[ ≡ (3/8)(μ/m)1/2(μv2/2Z2Ry)−1/2] is the collision parameter, Q⊥(≡ q⊥aZ) is the scaled transverse momentum transfer, and is the zeroth-order Bessel. As shown in Equations (14) and (15), the nonthermal shielding effects on the optically forbidden–forbidden
and allowed–allowed
transitions are explicitly indicated in the denominators of the integrands.
5. NONTHERMAL SHIELDING EFFECTS
Since the influence of nonthermal shielding on the collision cross section would be defined by , the nonthermal shielding function
for the optically allowed–allowed transition is represented by
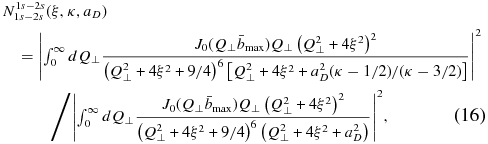
where for the forbidden–forbidden
transition is given by the position of the first-peak, i.e.,
. Additionally, the nonthermal shielding function
for the optically allowed–allowed transition is found to be
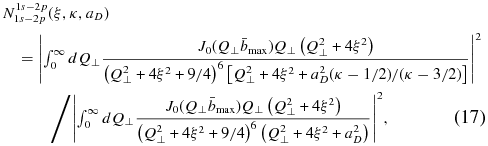
where for the allowed–allowed
transition is found to be
. Recently, the experimental momentum transfer collision between the electron and an atom in molecules has been investigated (Vos 2010). Hence, the nonthermal shielding effect on the ion–molecule collision (Dugan & Magee 1973), molecule–molecule collision (Shao et al. 2009), reactive atom–molecule collision, and electron–molecule collision (Sun & Dalgargo 1994; Hwang et al. 1996; Khristenko et al. 1998) in astrophysical Lorentzian plasmas will also be treated elsewhere using the semiclassical trajectory method. Hence, the excitation rate coefficients
in astrophysical Lorentzian plasmas would be obtained by the total excitation cross sections
(Equation (3)) and the Lorentzian distribution function fL(v; κ) (Equation (1)) in the following form:
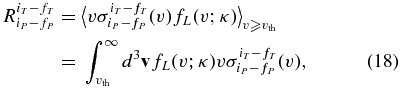
where the bracket 〈〉 denotes the ensemble average with the plasma distribution function, and vth stands for the threshold velocity of the projectile electron for the excitation process. Since the excitation rate coefficients are extremely important in plasmas, Equations (13) and (14) would be useful in investigating the discrete emission spectra due to the collisional excitation processes including the influence of nonthermal shielding in various astrophysical plasmas. The detailed discussion on the influence of polarization plasma effects has been obtained on the frequency of inelastic atomic transitions (Vinogradov & Shevelko 1976).
Figures 1 and 2 represent the momentum transfer-dependent effective projectile charge numbers for the optically forbidden (1s → 2s) and allowed (1s → 2p) transitions as functions of the scaled momentum transfer Q for various values of the spectral index κ of the nonthermal Lorentzian plasma. As shown in these figures, it is found that the nonthermal shielding effects suppress the effective projectile charge numbers
for both the optically allowed and forbidden transitions. It is also found that the influence of nonthermal shielding increases the critical momentum transfer value for the maximum effective projectile charge number
. It should be noted that the influence of non-thermal shielding on the effective projectile charge number
for the optically allowed (1s → 2p) transition is found to be greater than the influence on
for the optically forbidden (1s → 2s) transition. Additionally, we have found that the nonthermal shielding effects on the effective projectile charge numbers
for the optically forbidden (1s → 2s) and allowed (1s → 2p) transitions decrease with an increase of the Debye length, i.e., decrease of aD. Figure 3 shows the scaled differential cross section
as a function of the scaled impact parameter
for the optically forbidden–forbidden
transition in astrophysical Lorentzian plasmas owing to the ion–ion collisional excitation for a high-collision energy ξ = 0.03 for various values of the spectral index κ. From this figure, it is found that the influence of nonthermal shielding reduces the forbidden–forbidden
transition cross section near the first-peak region. However, it is interesting to note that the nonthermal shielding effect on the second peak of the differential cross section is almost negligible. We can expect that the existence of the node of the differential cross section would be caused by the physical characteristics of the 2s atomic wave function since the 2s radial wave function is orthogonal to the 1s radial wave function and the orthogonality produces the node and double peaks in the 2s radial wave function. It is also found that the maximum peak position of the differential cross section has receded from the target nucleus with increasing spectral index. Figure 4
represents the surface plot of the nonthermal shielding function for the optically forbidden–forbidden transition as a function of the scaled reciprocal Debye length aD and spectral index κ. From this figure, it is found that the nonthermal shielding effect on the forbidden–forbidden
transition increases with an increase of the reciprocal Debye length aD. Hence, the nonthermal character of the Lorentzian plasma would be more easily figured out in dense Lorentzian plasmas. Figure 5 represents the scaled differential cross section
as a function of the scaled impact parameter
for the optically forbidden–forbidden
transition in Lorentzian plasmas for an intermediate-collision energy ξ = 0.3 for various values of the spectral index κ. As it can be
seen, it is found that the second peak of the differential cross section has been disappeared in intermediate-collision energies. In addition, it is found that the influence of nonthermal shielding on the optically forbidden–forbidden transition decreases with a decrease of the collision energy. Then, the nonthermal character of the Lorentzian plasma would be more easily investigated in high-energy ion–ion collisions. Figure 6 shows the scaled differential cross section as a function of the scaled impact parameter
for the optically allowed–allowed
transition in Lorentzian plasmas owing to the ion–ion collisional excitation for a high-collision energy ξ = 0.03 for various values of the spectral index κ. As we expected from the case of the optically allowed–allowed transition, it is found that the nonthermal shielding effect strongly suppresses the whole range of the differential cross section for the optically allowed–allowed
transition. It is also found that the reaction range of the ion–ion collisional excitation in nonthermal Lorentzian plasmas is smaller than that in thermal Maxwellian plasmas. From Figures 3 and 6, the influence of nonthermal shielding on the optically allowed–allowed
transition is found to be greater than the influence on the optically forbidden–forbidden
transition. Figure 7 represents the surface plot of the nonthermal shielding function
for the optically allowed–allowed transition as a function of the scaled reciprocal Debye length aD and spectral index κ. As shown in this figure, it is also found that the nonthermal shielding effect on the allowed–allowed
transition increases with increasing reciprocal Debye length aD. Figure 8 shows the scaled differential cross section as a function of the scaled impact parameter
for the optically allowed–allowed
transition in Lorentzian plasmas for an intermediate-collision energy ξ = 0.3 for various values of the spectral index κ. As shown in this figure, it is found that the nonthermal shielding effect on the optically allowed–allowed transition decreases with decreasing collision energy in astrophysical nonthermal plasmas. In Table 1, the optically forbidden–forbidden
total transition cross sections
in units of
due to the hydrogen–hydrogen collisional excitations in astrophysical Lorentzian plasmas are given. In Table 2, the optically allowed–allowed
total transition cross sections
in units of
due to the hydrogen–hydrogen collisional excitations in astrophysical Lorentzian plasmas are given. In Table 3, the excitation rate coefficients
in units of R0[ ≡ (Z2Ryμ/2πm2)1/2(3aZ/8)2 = 4.15550 × 10−9(cm3 s−1)] for the optically forbidden–forbidden
transition are given in astrophysical Lorentzian plasmas due to the hydrogen–hydrogen collisional excitations for
for various values of the spectral index κ when aD = 0.1. In Table 4, the excitation rate coefficients
in units of R0[ ≡ (Z2Ryμ/2πm2)1/2(3aZ/8)2 = 4.15550 × 10−9(cm3s−1)] for the optically allowed–allowed
transition are given in astrophysical Lorentzian plasmas due to the hydrogen–hydrogen collisional excitations for
for various values of the spectral index κ when aD = 0.1.
Figure 1. Momentum transfer-dependent effective projectile charge numbers for the optically forbidden (1s → 2s) and allowed (1s → 2p) transitions as functions of the scaled momentum transfer Q for aD = 0.1. The solid line represents
for κ = 1.55. The dashed line represents
for κ = 6. The dotted line represents
for κ = 1.55. The dot-dashed line represents
for κ = 6.
Download figure:
Standard image High-resolution imageFigure 2. Momentum transfer-dependent effective projectile charge numbers for the optically forbidden (1s → 2s) and allowed (1s → 2p) transitions as functions of the scaled momentum transfer Q for aD = 0.05. The solid line represents
for κ = 1.55. The dashed line represents
for κ = 6. The dotted line represents
for κ = 1.55. The dot-dashed line represents
for κ = 6.
Download figure:
Standard image High-resolution imageFigure 3. Scaled differential cross section as a function of the scaled impact parameter
for the optically forbidden–forbidden
transition for a high-collision energy ξ = 0.03 and aD = 0.1. The solid line represents the case of κ = 1.6. The dashed line represents the case of κ = 2. The dotted line represents the case of κ → ∞, i.e., Maxwellian case.
Download figure:
Standard image High-resolution imageFigure 4. Surface plot of the nonthermal shielding function for the optically forbidden–forbidden transition as a function of the scaled reciprocal Debye length aD and spectral index κ for ξ = 0.03.
Download figure:
Standard image High-resolution imageFigure 5. Scaled differential cross section as a function of the scaled impact parameter
for the optically forbidden–forbidden
transition for an intermediate-collision energy ξ = 0.3 and aD = 0.1. The solid line represents the case of κ = 1.6. The dashed line represents the case of κ = 2. The dotted line represents the case of κ → ∞, i.e., Maxwellian case.
Download figure:
Standard image High-resolution imageFigure 6. Scaled differential cross section for the optically allowed–allowed
transition for a high-collision energy ξ = 0.03 and aD = 0.1. The solid line represents the case of κ = 1.6. The dashed line represents the case of κ = 2. The dotted line represents the case of κ → ∞, i.e., Maxwellian case.
Download figure:
Standard image High-resolution imageFigure 7. Surface plot of the nonthermal shielding function for the optically allowed–allowed transition as a function of the scaled reciprocal Debye length aD and spectral index κ for ξ = 0.03.
Download figure:
Standard image High-resolution imageFigure 8. Scaled differential cross section for the optically allowed–allowed
transition for an intermediate-collision energy ξ = 0.3 and aD = 0.1. The solid line represents the case of κ = 1.6. The dashed line represents the case of κ = 2. The dotted line represents the case of κ → ∞, i.e., Maxwellian case.
Download figure:
Standard image High-resolution imageTable 1. Optically Forbidden–Forbidden Total Transition Cross Sections
in Astrophysical Lorentzian Plasmas
κ Spectral Index | ![]() | |
---|---|---|
σ0[ = 8.79735 × 10−17(cm2)] | ||
ξ = 0.03 | ξ = 0.3 | |
1.6 | 0.00002236 | 0.00223135 |
2 | 0.00003186 | 0.00270055 |
3 | 0.00003364 | 0.00279472 |
4 | 0.00003398 | 0.00281394 |
5 | 0.00003412 | 0.00282224 |
6 | 0.00003420 | 0.00282687 |
7 | 0.00003425 | 0.00282982 |
8 | 0.00003429 | 0.00283186 |
9 | 0.00003431 | 0.00283337 |
10 | 0.00003433 | 0.00283451 |
Download table as: ASCIITypeset image
Table 2. Optically Allowed–Allowed Total Transition Cross Sections
in Astrophysical Lorentzian Plasmas
κ Spectral | ![]() | |
---|---|---|
Index | σ0[ = 8.79735 × 10−17(cm2)] | |
ξ = 0.03 | ξ = 0.3 | |
1.6 | 0.0005053 | 0.0202450 |
2 | 0.0009601 | 0.0282981 |
3 | 0.0011381 | 0.0296662 |
4 | 0.0011848 | 0.0299495 |
5 | 0.0012064 | 0.0300722 |
6 | 0.0012189 | 0.0301407 |
7 | 0.0012267 | 0.0301844 |
8 | 0.0012327 | 0.0302148 |
9 | 0.0012369 | 0.0302371 |
10 | 0.0012401 | 0.0302541 |
Download table as: ASCIITypeset image
Table 3. Optically Forbidden–Forbidden Excitation Rate Coefficients
in Astrophysical Lorentzian Plasmas
κ Spectral | ![]() |
---|---|
Index | R0[ = 4.15550 × 10−9(cm3s−1)] |
![]() | |
1.6 | 2.52834 × 10−12 |
2 | 9.69654 × 10−13 |
3 | 1.46496 × 10−14 |
4 | 3.42281 × 10−16 |
5 | 1.75303 × 10−17 |
6 | 2.24806 × 10−18 |
7 | 6.35617 × 10−19 |
8 | 2.92579 × 10−19 |
9 | 1.75009 × 10−19 |
10 | 1.21151 × 10−19 |
Download table as: ASCIITypeset image
Table 4. Optically Allowed–Allowed Excitation Rate Coefficients
in Astrophysical Lorentzian Plasmas
κ Spectral | ![]() |
---|---|
Index | R0[ = 4.15550 × 10−9 (cm3s−1)] |
![]() | |
1.6 | 1.47400 × 10−12 |
2 | 4.54588 × 10−13 |
3 | 3.13722 × 10−15 |
4 | 2.93061 × 10−17 |
5 | 4.73949 × 10−19 |
6 | 1.43285 × 10−20 |
7 | 8.84043 × 10−22 |
8 | 1.16847 × 10−22 |
9 | 2.98803 × 10−23 |
10 | 1.20055 × 10−23 |
Download table as: ASCIITypeset image
6. CONCLUSIONS
In this paper, we investigated the influence of nonthermal shielding on the optically allowed and forbidden anti-screening excitation channels for ion–ion collisions in astrophysical Lorentzian plasmas. We employed the semiclassical trajectory approximation and the effective interaction Hamiltonian to obtain the transition amplitudes for the ion–ion collisional allowed–allowed and forbidden–forbidden excitations as functions of the impact parameter, collision energy, Debye radius, and spectral index. We also obtained the momentum transfer-dependent effective projectile charge in Lorentzian plasmas as a function of the momentum transfer, Debye radius, and spectral index. In this work, we have found that the nonthermal shielding effect suppresses the effective projectile charge numbers for both the optically allowed and forbidden transitions in Lorentzian plasmas. It is also found that the nonthermal shielding effect on the effective projectile charge number
for the optically allowed transition is greater than the effect on
for the optically forbidden transition. We have found that the nonthermal shielding effect reduces the forbidden–forbidden
transition cross section near the first-peak region; however, the nonthermal shielding effect on the second peak of the differential cross section is almost negligible. It is also found that the nonthermal shielding effect on the forbidden–forbidden transition increases with an increase of the reciprocal Debye length aD. It is also found that the influence of nonthermal shielding on the optically forbidden–forbidden transition decreases with decreasing collision energy. Hence, we can expect that the nonthermal property of the astrophysical Lorentzian plasma would be more easily investigated in high-energy ion–ion collisions. For the optically allowed–allowed
transition, the influence of nonthermal shielding strongly suppresses the differential cross section in whole domains. In this work, we have also found that the nonthermal shielding effect on the optically allowed–allowed
transition is greater than the effect on the optically forbidden–forbidden
transition. Additionally, we have found that the reaction ranges of the ion–ion collisional excitations in astrophysical nonthermal Lorentzian plasmas are smaller than those in thermal Maxwellian plasmas. As in the forbidden–forbidden transition, it is found that the nonthermal shielding effect on the allowed–allowed
transition increases with increasing reciprocal Debye length aD. We have also found that the influence of nonthermal shielding on the optically allowed–allowed transition decreases with a decrease of the collision energy. From this work, we have found that the nonthermal shielding effect plays a crucial role in the optically allowed–allowed and forbidden–forbidden anti-screening channels for ion–ion collisional excitations in astrophysical Lorentzian plasmas. These results would provide useful information on the ion–ion collisional anti-screening excitations and also on diagnostics of the physical conditions in astrophysical nonthermal plasmas.
Y.-D.J. gratefully acknowledges Prof. W. Roberge for useful discussions and warm hospitality while visiting the Department of Physics, Applied Physics, and Astronomy at Rensselaer Polytechnic Institute (RPI). This research was initiated while one of the authors (Y.-D.J.) was affiliated with RPI as a visiting professor. This paper is dedicated to the late Prof. R. J. Gould in memory of exciting and stimulating collaborations on atomic and molecular processes in astrophysical plasmas. This research was supported by the Basic Science Research Program through the National Research Foundation of Korea (NRF) funded by the Ministry of Education, Science, and Technology (Grant No. 2012-001493).