ABSTRACT
Using state-of-the-art computational methodologies, we predict a set of reliable rotational and torsional parameters for ethyl mercaptan and dimethyl sulfide monosubstituted isotopologues. This includes rotational, quartic, and sextic centrifugal-distortion constants, torsional levels, and torsional splittings. The accuracy of the present data was assessed from a comparison to the available experimental data. Generally, our computed parameters should help in the characterization and the identification of these organo-sulfur molecules in laboratory settings and in the interstellar medium.
Export citation and abstract BibTeX RIS
1. INTRODUCTION
Given the improved capabilities of new astronomical observatories in terms of spectral coverage and spatial resolution, the corresponding surveys cover large frequency ranges and can contain many unidentified lines. The latter features usually correspond to "new" molecules and/or rare isotopologues of previously detected species. The "cleaning" of the corresponding spectral confusion implies the full assignment of astronomical spectra, which in turn requires a complete spectroscopic characterization of all of the involved species at different temperatures.
Organic molecules can be detected in low-energy, excited vibrational states in hot molecular cores through their rotational spectra. Furthermore, nonrigid species such as ethyl mercaptan and dimethyl sulfide present internal rotation motions restricted by energy barriers. These motions can produce splitting of the ground and low-excited vibrational levels through the tunneling effect. Their complete characterization at low temperatures requires analysis of the vibrational spectrum in the far-infrared region. Unfortunately, a large number of detectable molecules are not well characterized. For many species, although accurate spectroscopic data are available, they are limited to the vibrational ground state and to the most abundant isotopologues. The effect of temperature and the fact that isotopic composition can be very different from one extraterrestrial source to another are often not considered. However, species containing less abundant isotopes or molecules with populated low vibrational states can play important chemical roles in many sources. An example is provided by methyl formate (a nonrigid molecule), for which investigations have been reported for the rotational spectrum in the vibrational ground state for the main isotopic species (Brown et al. 1975; Churchwell & Winnewisser 1975) as well as for several isotopologues (Carvajal et al. 2009; Margulès et al. 2010; Tercero et al. 2012). Features due to the first excited torsional state have also been detected for the main isotopologue (Kobayashi et al. 2007; Demyk et al. 2008) and 13C-containing (Carvajal et al. 2010) isotopologues. The main isotopic species was also observed in the second excited torsional state (Takano et al. 2012).
State-of-the-art ab initio methods can be used to compute highly accurate spectroscopic constants, even for nonrigid molecules (Brites et al. 2008; Halvick et al. 2011; Puzzarini et al. 2014a; Senent et al. 2009, 2014). Today, these constants are known to provide the required accuracy to guide experimental investigations of rotational and far-infrared spectra. While experimental determinations for rare isotopic species can be hampered by their low natural abundance, computations provide the same accuracy for all isotopologues. Therefore, quantum-chemical calculations are well suited to support and complement experimental and astronomical studies that also involve rare isotopic species. Examples can be found in investigations of the main isotopologues and several other isotopologues containing D and 13C of dimethyl-ether (DME; Senent et al. 2012; Carvajal et al. 2012, 2014) or propane (Villa et al. 2013).
Recently, we conducted a study of the main isotopic species of dimethyl sulfide (DMS) and ethyl mercaptan (ETSH) at low temperatures (Senent et al. 2014) considering their potential astrophysical relevance. In particular, the latter molecule was considered to be a potential detectable sulfur organic compound based on different arguments: the importance of sulfur chemistry in the interstellar medium (Charnley 1997) and because the O-analog of ethyl mercaptan, ethanol, is a well-known astrophysical molecule. We should consider that usually, with few exceptions (Cernicharo et al. 1987), the detection of S-bearing species follows the detection of corresponding O-analogs. The expectations of astrophysicists were recently satisfied when an exhaustive search led to the detection of ethyl-mercaptan in Orion in 2014 (Kolesniková et al. 2014). For many years, methyl mercaptan was the only sulfur nonrigid molecule detected in astrophysical sources (Linke et al. 1979).
Differences in their formation processes have made determination of the isotopic abundances of different sources particularly interesting as a means of investigating interstellar and galactic evolution (Mauersberger et al. 2004). In addition, fluctuations of the relative isotopic abundances are tools for identifying chemical, geophysical, and biological processes (Canfield 2001; Farquhar & Wing 2003; Mauersberger et al. 2004). Hence, relative isotopic abundances have been estimated for meteorites, the moon, cosmic rays, and stars (Mauersberger et al. 2004). There is some evidence demonstrating that isotope yields follow different synthesis procedures. Whereas 32S, 34S, and 33S are the primary products of oxygen burning in a star, 36S is produced when the primary sulfur isotopes capture neutrons during helium and carbon burning (Mauersberger et al. 1996).
Considering their potential importance, in the present work, we investigated several rare monosubstituted isotopologues of ethyl mercaptan and dimethyl sulfide containing either a rare S-isotope or deuterium. Sulfur, the tenth most abundant cosmological element, presents four stable isotopes 32S, 34S, 33S, and 36S whose abundance ratios in the solar system were estimated to be 95.02%, 4.21%, 0.75%, and 0.021% (Anders & Grevesse 1989), respectively. Their relative abundances in Orion KL were determined to be 32S/34S = 20 ± 6, 32S/33S = 75 ± 29 (Tercero et al. 2010a, 2010b). The first molecule containing 36S,C36S, was detected in Galactic molecular hot cores by Mauersberger et al. (1996), who estimated a relative abundance of 34S/36S = 115 ± 17, which is smaller than that in the solar system. This ratio is consistent with what was later determined by Mauersberger et al. (2004), 107 ± 15, in the carbon star IRC+ 10216 based on the detection of the rotational transitions of C36S and Si36S. Moreover, several S-containing molecules were detected in the ISM raging from diatomics (e.g. NS, SO, and SH) to more complex small organic molecules (e.g. C2S, H2S, OCS, C3S, H2CS, HSCN, HNCS, CH3CS, etc.; Ziurys 2006; Millar et al. 1986; Irvine et al. 1988; Goldsmith et al. 1981; Minh et al. 1991; Frerking et al. 1979; Linke et al. 1979). Si36S is the first compound containing 36S detected in a star (Mauersberger et al. 2004).
In the present work, by following the procedures of Senent et al. (2014), we have investigated the 34S-, 36S-, 33S-, and deuterium-containing ETSH and DMS species, considering them not only in the vibrational ground state, but also in their excited torsional states because at the temperature of the hot molecular cores, these excited states and their splittings can be populated. Hence, we provide a detailed spectroscopic characterization of these S-containing molecules and isotopologues to help their detection in the ISM. Thus, the aim of this paper is to predict the isotopic substitution effect on the rotational and torsional parameters. To assess their accuracy, the computed properties were compared with the available experimental data, in particular, for the main isotopologues (Senent et al. 2014). While for the latter we refer to Senent et al. (2014) for an account on previous theoretical and experimental studies, here we mention that for other isotopic species, only a few studies are available (Hayashi et al. 1989; Schmidt & Quade 1975; Wolff & Szydlowski 1985; Manocha et al. 1973; Kretschmer et al. 1995; Kolesniková et al. 2014).
2. METHODOLOGY
In this study, we followed the methodologies described in Puzzarini et al. (2010), Puzzarini (2013), and Senent (1998a, 1998b, 2001). In particular, we refer interested readers to Puzzarini et al. (2010) and Puzzarini (2013) for rotational spectroscopy and to Senent (1998a, 1998b, 2001) for torsional analysis. In particular, concerning the spectroscopic characterization of ETSH and DMS, all computational details can be found in Senent et al. (2014). In the following, only a brief summary is provided. Here, we also point out that DMS has two equivalent methyl groups leading to nine equivalent minima in the potential energy surface (PES), while ETSH has a unique methyl group which is responsible for three equivalent minima. In addition, for the latter, a second torsional coordinate, the thiol torsion (SH torsion), leads to two conformers, the gauche and trans forms. Then, the coupling of the two torsional motions in ETSH (methyl and thiol torsions) generates a PES with nine minima (for further details, see Senent et al. 2014).
2.1. Rotational Spectroscopy
To obtain accurate equilibrium rotational constants, the equilibrium structures of ETSH and DMS were determined by means of a composite scheme (see Senent et al. 2014) which is based on additivity at an energy-gradient level (Heckert et al. 2005, 2006) and employs the coupled-cluster singles and doubles approximation (CCSD) augmented by a perturbative treatment of triple excitations [CCSD(T)] (Raghavachari et al. 1989) in conjunction with correlation-consistent basis sets, cc-p(C)VnZ (n = T, Q, 5) (Dunning 1989; Woon & Dunning 1995). Second, to derive vibrational ground-state and torsional-excited-state rotational constants, the equilibrium rotational constants were corrected for vibrational effects with the corresponding corrections being obtained by means of second-order vibrational perturbation theory (VPT2; Mills 1972) at the MP2/cc-pVTZ, CCSD/cc-pVTZ, and CCSD(T)/cp-VTZ levels (for details, see Senent et al. 2014), where MP2 stands for the Møller–Plesset theory to the second order (Møller & Plesset 1934). These calculations implied the evaluation of cubic force fields, and therefore they also allowed us to determine quartic and sextic centrifugal-distortion constants. Different levels of theory were considered in order to verify the cheapest one while still providing reliable and accurate results. All of these calculations were carried out with the quantum-chemical CFOUR program package (2012).
To further improve the predictive capabilities of our computed parameters (i.e., rotational and centrifugal-distortion constants), an empirical scaling procedure was employed for the ground-state parameters. For a generic parameter X, the latter procedure is based on multiplying the computed value of X for a monosubstituted isotopologue (denoted by the superscript iso) for the corresponding experiment/theory ratio for the main (32S-containing) isotopic species (denoted by the superscript main):

where scal, exp, and calc denote the scaled, experimental, and quantum-chemically calculated values for X, respectively. This approach is extensively used in the field of rotational spectroscopy, and its validity has been discussed, for example, in Puzzarini et al. (2012). For gauche-ETSH, for which a complete set of experimental rotational and quartic centrifugal-distortion constants is available for the 34S-containing species, the latter was used for the reference isotopologue in order to derive the scaled parameters for the main isotopic species.
2.2. Torsional Analysis
For the analysis of the vibrational spectrum in the far-infrared region, we have performed a torsional analysis following the theoretical methodology described in our previous paper (Senent et al. 2014). The energy levels are determined variationally by solving the following two-dimensional Hamiltonian:
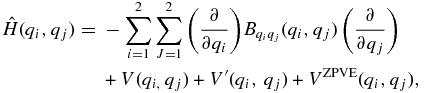
which depends on two independent coordinates qi and qj. For ETSH, qi and qj are identified as the CH3 torsion (θ) and the SH torsion (α), respectively. In DMS, both coordinates correspond to methyl internal rotations (θ1 and θ2). V(qi, qj) represents the two-dimensional potential energy surface (2D-PES). VZPVE(qi, q2), Bqiqj, and V ' (qi, qj) denote the zero-point vibrational energy correction, the kinetic energy parameters, and the Podolsky pseudopotential, respectively. For their definition, the readers are referred to Senent (1998a, 1998b).
Since the 2D-PES is isotopically invariant, here we use the surfaces generated in our previous study to treat the main isotopologue. These surfaces were obtained from the total electronic energies calculated at the CCSD(T)/aug-cc-pVTZlevel of theory (Kendall et al. 1992) for a number NS of selected geometries defined for different values of the independent coordinates (NS = 26 for ETSH and NS = 7 for DMS). For each point of the PES, the remaining 3Na-6-n internal coordinates (Na = number of atoms, n = 2 dihedral angles) were optimized at the CCSD/aug-cc-pVTZ level.
VZPVE(qi, q2), Bqiqj, and V '(qi, qj) are, however, isotopically dependent. For each NS structure and for each isotopologue, Bqiqj and V '(qi, qj) were determined using the code ENEDIM, also employed in the variational calculation of the energy levels. VZPVE(qi, q2) was calculated within the harmonic approximation at the MP2/aug-cc-pVTZ level using the Gaussian 09 package. For all details, the reader is referred to Senent et al. (2014).
3. RESULTS
3.1. Rotational Parameters
The computed vibrational-ground-state rotational and centrifugal-distortion constants of the isotopologues considered for gauche-ETSH, trans-ETSH, and DMS are collected in Tables 1–3, with the Watson's A reduction in the I r representation (Watson 1977) taken into consideration. Tables 1 and 2 summarize in detail the spectroscopic parameters obtained for the isotopologues considered for gauche-ETSH and trans-ETSH. Table 3 reports the results for DMS isotopic species. While in Tables 1–3 we only report our best-computed values (i.e., those based on CCSD(T) calculations), the CCSD parameters scaled according to Equation (1), and the available experimental data, the complete set of our results is supplied in our tables. In particular, we present the comparison of the MP2, CCSD, and CCSD(T) results.
Table 1. Computed, Scaled, and Experimental Rotational Parametersa for gauche-ethyl Mercaptan
gauche-CH3CH232SH | ||||||
---|---|---|---|---|---|---|
Parameter | Calculated | Scalede | Experiment Kolesniková et al. (2014) | |||
MP2b | CCSDc | CCSD(T)d | CCSD | 0+ | 0− | |
A0 | 28783.924 | 28779.449 | 28775.409 | 28748.096 | 28747.4104(66) | 28747.2715(65) |
B0 | 5300.793 | 5300.891 | 5298.833 | 5295.107 | 5295.1422(36) | 5295.0008(36) |
C0 | 4850.955 | 4850.923 | 4849.312 | 4845.829 | 4845.9421(36) | 4845.9689(36) |
ΔJ | 3.362 | 3.287 | 3.364 | 3.328 | 3.326369(20) | 3.323582(20) |
ΔJK | −20.330 | −19.571 | −19.809 | −18.402 | −18.39280(60) | −18.35859(60) |
ΔK | 205.641 | 205.263 | 206.297 | 206.932 | 204.1591(82) | 203.9217(81) |
δJ | 0.537 | 0.518 | 0.534 | 0.514 | 0.514429(12) | 0.513170(14) |
δK | 10.016 | 9.592 | 9.960 | 8.482 | 8.781(12) | 8.555(12) |
ΦJ | −0.0027 | −0.0027 | −0.0029 | 0.0029872(28) | 0.0029225(31) | |
ΦJK | −0.1125 | −0.1182 | −0.1201 | 0.0790(13) | 0.0661(13) | |
ΦKJ | 0.9420 | 1.0045 | 0.9973 | −1.3341(45) | −1.2955(44) | |
ΦK | −3.3603 | −3.6061 | −3.5997 | 6.341(48) | 5.171(46) | |
ϕJ | −0.0011 | −0.0011 | −0.0012 | 0.0012107(15) | 0.0011805(16) | |
ϕJK | −0.0597 | −0.0631 | −0.0647 | 0.04561(63) | 0.04326(65) | |
ϕK | 1.9055 | 1.9526 | 2.0270 | 5.858(95) | 4.825(97) | |
gauche-CH3CH234SH | ||||||
Parameter | Calculated | Scalede | Experiment Kolesniková et al. (2014) | |||
MP2b | CCSDc | CCSD(T)d | CCSD | 0+ | 0− | |
A0 | 28745.294 | 28741.143 | 28737.106 | 28709.078 | 28709.699(24) | 28708.964(26) |
B0 | 5181.142 | 5181.156 | 5179.154 | 5175.469 | 5175.5685(36) | 5175.4383(36) |
C0 | 4749.762 | 4749.706 | 4748.131 | 4744.843 | 4744.7050(36) | 4744.7310(36) |
ΔJ | 3.215 | 3.143 | 3.217 | 3.179 | 3.18351(18) | 3.18084(18) |
ΔJK | −19.798 | −19.064 | −19.290 | −17.899 | −17.9512(15) | −17.8994(14) |
ΔK | 204.658 | 204.289 | 205.308 | 203.072 | 206.8(14) | 205.1(14) |
δJ | 0.502 | 0.484 | 0.499 | 0.480 | 0.481132(82) | 0.480129(71) |
δK | 9.628 | 9.214 | 9.569 | 8.326 | 8.271(34) | 8.024(35) |
ΦJ | −0.0024 | −0.0024 | −0.0025 | |||
ΦJK | −0.1066 | −0.1111 | −0.1129 | |||
ΦKJ | 0.8831 | 0.9400 | 0.9328 | |||
ΦK | −3.1091 | −3.4068 | −3.4003 | |||
ϕJ | −0.0010 | −0.0010 | −0.0011 | |||
ϕJK | −0.0545 | −0.0577 | −0.0592 | |||
ϕK | 1.8209 | 1.8821 | 1.9542 | |||
gauche-CH3CH233SH | gauche-CH3CH236SH | |||||
Parameter | Calculated | Scaled | Calculated | Scaled | ||
CCSDc | CCSD(T)d | CCSDe | CCSDc | CCSD(T)d | CCSDe | |
A0 | 28759.688 | 28755.646 | 28727.602 | 28707.028 | 28702.999 | 28675.000 |
B0 | 5239.310 | 5237.282 | 5233.559 | 5073.263 | 5071.311 | 5067.6946 |
C0 | 4798.905 | 4797.313 | 4793.991 | 4658.229 | 4656.686 | 4653.4594 |
ΔJ | 3.210 | 3.288 | 3.247 | 3.010 | 3.087 | 3.045 |
ΔJK | −19.208 | −19.541 | −18.034 | −18.744 | −18.833 | −17.599 |
ΔK | 204.239 | 205.786 | 203.023 | 204.271 | 204.428 | 203.054 |
δJ | 0.500 | 0.515 | 0.496 | 0.454 | 0.469 | 0.451 |
δK | 9.402 | 9.758 | 8.496 | 8.846 | 9.222 | 7.994 |
ΦJ | −0.0026 | −0.0022 | ||||
ΦJK | −0.1145 | −0.1048 | ||||
ΦKJ | 0.9711 | 0.8833 | ||||
ΦK | −3.5034 | −3.2284 | ||||
ϕJ | −0.0011 | −0.0009 | ||||
ϕJK | −0.0603 | −0.0531 | ||||
ϕK | 1.9160 | 1.8202 | ||||
gauche-CH3CH232SD | ||||||
Parameters | Calculated | Scaled | Experiment Schmidt & Quade (1975) | |||
CCSDc | CCSD(T)d | CCSDe | ||||
A0 | 26092.013 | 26087.580 | 26062.904 | 26064.003 | ||
B0 | 5195.122 | 5193.214 | 5189.419 | 5190.090 | ||
C0 | 4773.556 | 4772.141 | 4768.672 | 4768.620 | ||
ΔJ | 3.378 | 3.521 | 3.416 | |||
ΔJK | −13.104 | −13.931 | −12.303 | |||
ΔK | 140.806 | 143.593 | 139.967 | |||
δJ | 0.585 | 0.626 | 0.580 | |||
δK | 13.190 | 22.893 | 11.919 | |||
ΦJ | −0.0102 | |||||
ΦJK | −0.0664 | |||||
ΦKJ | 0.8960 | |||||
ΦK | −4.1109 | |||||
ϕJ | −0.0046 | |||||
ϕJK | −0.1203 | |||||
ϕK | 4.7852 |
Notes. aRotational constants in MHz; quartic and sextic centrifugal-distortion constants in kHz and Hz, respectively. Watson A-reduction in the I r representation is used. bEquilibrium constants corresponding to the best-estimated equilibrium structure (see text; Senent et al. 2014). Vibrational corrections to rotational constants, and quartic and sextic centrifugal-distortion constants at the MP2/cc-pVTZ level. cEquilibrium constants corresponding to the best-estimated equilibrium structure (see text; Senent et al. 2014). Vibrational corrections to rotational constants, and quartic and sextic centrifugal-distortion constants at the CCSD/cc-pVTZ level. dEquilibrium constants corresponding to the best-estimated equilibrium structure (see text; Senent et al. 2014). Vibrational corrections to rotational constants, and quartic and sextic centrifugal-distortion constants at the CCSD(T)/cc-pVTZ level. eFor 32S, the experimental data of the 34S-containing isotopologue were used for the scaling procedure; for 34S, 33S, 36S, and D, the experimental values of 32S were employed. For experiment, the averaged 0+ and 0− values are used.
Table 2. Computed, Scaled, and Experimental Rotational Parametersa for trans-ethyl Mercaptan
trans-CH3CH232SH | ||||
---|---|---|---|---|
Parameters | Calculated | Experiment Kolesniková et al. (2014) | ||
MP2b | CCSDc | CCSD(T)d | ||
A0 | 28460.983 | 28460.659 | 28451.975 | 28416.7604 (18) |
B0 | 5492.059 | 5491.347 | 5489.912 | 5485.77901 (15) |
C0 | 4886.820 | 4886.618 | 4885.345 | 4881.81770 (15) |
ΔJ | 3.795 | 3.679 | 3.767 | 3.83217 (27) |
ΔJK | −23.558 | −22.847 | −23.107 | −22.4549 (58) |
ΔK | 196.710 | 197.969 | 198.873 | 210.03 (18) |
δJ | 0.646 | 0.617 | 0.634 | 0.65664 (11) |
δK | 6.091 | 5.807 | 5.967 | 7.342 (20) |
ΦJ | −0.0116 | −0.0120 | −0.0130 | −0.00086 (17) |
ΦJK | −0.0415 | −0.0467 | −0.0423 | 0.367 (18) |
ΦKJ | 0.9175 | 1.0659 | 1.0607 | −2.717 (106) |
ΦK | −4.6243 | −5.3975 | −5.4647 | 26.7 (80) |
ϕJ | −0.0057 | −0.0059 | −0.0064 | −0.000935 (91) |
ϕJK | −0.2014 | −0.2136 | −0.2265 | −0.164 (26) |
ϕK | −1.0809 | −1.2489 | −1.3450 | 28.89 (97) |
trans-CH3CH234SH | ||||
Parameters | Calculated | Scaled | ||
MP2b | CCSDc | CCSD(T)d | CCSDe | |
A0 | 28374.015 | 28373.744 | 28365.113 | 28329.979 |
B0 | 5373.179 | 5372.489 | 5371.085 | 5367.041 |
C0 | 4789.981 | 4789.783 | 4788.536 | 4785.077 |
ΔJ | 3.659 | 3.541 | 3.634 | 3.688 |
ΔJK | −23.153 | −22.564 | −22.585 | −22.177 |
ΔK | 195.493 | 197.344 | 196.995 | 209.367 |
δJ | 0.613 | 0.585 | 0.602 | 0.622 |
δK | 5.948 | 5.624 | 5.868 | 7.110 |
ΦJ | −0.0105 | −0.0109 | −0.0117 | |
ΦJK | −0.0408 | −0.0457 | −0.0418 | |
ΦKJ | 0.8413 | 0.9788 | 0.9735 | |
ΦK | −4.2527 | −4.9684 | −5.0279 | |
ϕJ | −0.0051 | −0.0053 | −0.0057 | |
ϕJK | −0.1854 | −0.1966 | −0.2085 | |
ϕK | −0.9751 | −1.1294 | −1.2178 | |
trans-CH3CH233SH | ||||
Parameters | Calculated | Scaled | ||
CCSDc | CCSD(T)d | CCSDe | ||
A0 | 28415.795 | 28407.139 | 28371.966 | |
B0 | 5430.260 | 5428.841 | 5424.754 | |
C0 | 4836.877 | 4835.617 | 4832.125 | |
ΔJ | 3.610 | 3.699 | 3.761 | |
ΔJK | −22.716 | −22.838 | −22.326 | |
ΔK | 197.693 | 197.905 | 209.737 | |
δJ | 0.601 | 0.617 | 0.639 | |
δK | 5.716 | 5.917 | 7.227 | |
ΦJ | −0.0114 | −0.0123 | ||
ΦJK | −0.0462 | −0.0431 | ||
ΦKJ | 1.0204 | 1.0182 | ||
ΦK | −5.1738 | −5.2391 | ||
ϕJ | −0.0056 | −0.0060 | ||
ϕJK | −0.2048 | −0.2167 | ||
ϕK | −1.1869 | −1.3222 | ||
trans-CH3CH236SH | ||||
Parameters | Calculated | Scaled | ||
CCSDc | CCSD(T)d | CCSDe | ||
A0 | 28296.527 | 28287.940 | 28252.881 | |
B0 | 5265.097 | 5263.722 | 5259.759 | |
C0 | 4702.094 | 4700.871 | 4697.475 | |
ΔJ | 3.400 | 3.514 | 3.542 | |
ΔJK | −22.202 | −22.115 | −21.821 | |
ΔK | 196.451 | 195.316 | 208.420 | |
δJ | 0.553 | 0.573 | 0.588 | |
δK | 5.437 | 5.774 | 6.874 | |
ΦJ | −0.0099 | −0.0106 | ||
ΦJK | −0.0447 | −0.0422 | ||
ΦKJ | 0.9050 | 0.9026 | ||
ΦK | −4.6015 | −4.6568 | ||
ϕJ | −0.0048 | −0.0052 | ||
ϕJK | −0.1820 | −0.1926 | ||
ϕK | −1.0252 | −1.1520 | ||
trans-CH3CH232SD | ||||
Parameters | Calculated | Scaled | Experiment Schmidt & Quade (1975) | |
CCSDc | CCSD(T)d | CCSDe | ||
A0 | 27202.948 | 27194.339 | 27160.989 | 27155.91 |
B0 | 5308.668 | 5307.475 | 5303.285 | 5304.36 |
C0 | 4706.623 | 4705.557 | 4701.999 | 4702.60 |
ΔJ | 3.166 | 3.253 | 3.298 | |
ΔJK | −16.455 | −17.670 | −16.172 | |
ΔK | 147.484 | 154.696 | 156.469 | |
δJ | 0.524 | 0.541 | 0.557 | |
δK | 3.826 | 3.671 | 4.838 | |
ΦJ | −0.0417 | −0.0452 | ||
ΦJK | −0.0860 | −0.0814 | ||
ΦKJ | 5.2740 | 5.4727 | ||
ΦK | −24.3435 | −25.1609 | ||
ϕJ | −0.0208 | −0.0225 | ||
ϕJK | −0.6558 | −0.7025 | ||
ϕK | −6.3870 | −6.8136 |
Notes. aRotational constants in MHz; quartic and sextic centrifugal-distortion constants in kHz and Hz, respectively. Watson A-reduction in the I r representation is used. bEquilibrium constants corresponding to the best-estimated equilibrium structure (see text; Senent et al. 2014). Vibrational corrections to rotational constants, and quartic and sextic centrifugal-distortion constants at the MP2/cc-pVTZ level. cEquilibrium constants corresponding to the best-estimated equilibrium structure (see text; Senent et al. 2014). Vibrational corrections to rotational constants, and quartic and sextic centrifugal-ditortion constants at the CCSD/cc-pVTZ level. dEquilibrium constants corresponding to the best-estimated equilibrium structure (see text; Senent et al. 2014). Vibrational corrections to rotational constants, and quartic and sextic centrifugal-ditortion constants at the CCSD(T)/cc-pVTZ level. eEmpirically scaled parameters (see text) starting from those computed as at footnote c.
Table 3. Computed, Scaled, and Experimental Rotational Parametersa for Dimethyl-Sulfide
CH332SCH3 | |||||
---|---|---|---|---|---|
Parameter | Calculated | Experiment Vacherand et al. (1987) | Experiment Hayashi et al. (1989). | ||
MP2b | CCSDc | CCSD(T)d | |||
A0 | 17836.230 | 17832.456 | 17825.867 | 17810.0389(35) | 17809.734(8) |
B0 | 7630.756 | 7631.159 | 7629.422 | 7621.12253(110) | 7621.098(2) |
C0 | 5725.777 | 5725.526 | 5724.127 | 5717.76282(101) | 5717.769(2) |
ΔJ | 8.692 | 8.324 | 8.609 | 8.04258(118) | 8.06(4) |
ΔJK | −40.406 | −38.073 | −39.071 | −35.2214(103) | −35.45(43) |
ΔK | 140.456 | 138.442 | 139.591 | 139.572(49) | 140.88(165) |
δJ | 3.139 | 2.970 | 3.086 | 2.82209(21) | 2.84(2) |
δK | 3.635 | 3.396 | 3.440 | 3.8458(156) | 3.29(49) |
ΦJ | −0.0457 | −0.0485 | −0.0519 | ||
ΦJK | −0.0436 | −0.0571 | −0.0528 | −0.1152(138) | |
ΦKJ | 1.3772 | 1.5930 | 1.6203 | 0.462(82) | |
ΦK | −3.8297 | −4.4210 | −4.4775 | ||
ϕJ | −0.0227 | −0.0241 | −0.0258 | ||
ϕJK | −0.2395 | −0.2654 | −0.2759 | 0.2277(161) | |
ϕK | 0.8114 | 0.7648 | 0.7885 | ||
CH334SCH3 | |||||
Parameter | Calculated | Scaled | Experiment Hayashi et al. (1989). | ||
CCSDc | CCSDd | CCSDe | |||
A0 | 17420.928 | 17414.496 | 17399.028 | 17398.825(9) | |
B0 | 7631.361 | 7629.625 | 7621.324 | 7621.335(4) | |
C0 | 5682.329 | 5680.944 | 5674.624 | 5674.658(8) | |
ΔJ | 8.274 | 8.558 | 8.011 | 8.46(5) | |
ΔJK | −37.506 | −38.496 | −34.697 | −35.12(40) | |
ΔK | 134.375 | 135.486 | 135.472 | 17398.825(9) | |
δJ | 2.995 | 3.112 | 2.846 | ||
δK | 3.198 | 3.230 | 3.621 | ||
ΦJ | −0.0472 | ||||
ΦJK | −0.0411 | ||||
ΦKJ | 1.4401 | ||||
ΦK | −4.0653 | ||||
ϕJ | −0.0234 | ||||
ϕJK | −0.2445 | ||||
ϕK | 0.7414 | ||||
CH333SCH3 | |||||
Parameter | Calculated | Scaled | Experiment Kretschmer et al. (1995) | ||
CCSDc | CCSD(T)d | CCSDe | |||
A0 | 17620.725 | 17614.217 | 17598.574 | 17598.3002(14) | |
B0 | 7631.263 | 7629.527 | 7621.2264 | 7622.2643(24) | |
C0 | 5703.472 | 5702.080 | 5695.7379 | 5694.7067(24) | |
ΔJ | 8.582 | 8.582 | 8.034 | 8.115(32) | |
ΔJK | −38.776 | −38.776 | −34.955 | −35.36(16) | |
ΔK | 137.481 | 137.481 | 137.462 | 137.07(27) | |
δJ | 3.099 | 3.099 | 2.834 | 2.845(8) | |
δK | 3.335 | 3.335 | 3.728 | 3.63(25) | |
ΦJ | −0.0478 | ||||
ΦJK | −0.0488 | ||||
ΦKJ | 1.5135 | ||||
ΦK | −4.2362 | ||||
ϕJ | −0.0238 | ||||
ϕJK | −0.2546 | ||||
ϕK | 0.7530 | ||||
CH336SCH3 | |||||
Parameter | Calculated | Scaled | |||
CCSDc | CCSD(T)d | CCSDe | |||
A0 | 17050.624 | 17044.335 | 17029.190 | ||
B0 | 7631.541 | 7629.806 | 7621.504 | ||
C0 | 5642.268 | 5640.897 | 5634.618 | ||
ΔJ | 8.513 | 8.513 | 7.969 | ||
ΔJK | −37.977 | −37.977 | −34.235 | ||
ΔK | 131.786 | 131.786 | 131.768 | ||
δJ | 3.134 | 3.134 | 2.866 | ||
δK | 3.024 | 3.024 | 3.381 | ||
ΦJ | −0.0460 | ||||
ΦJK | −0.0276 | ||||
ΦKJ | 1.3083 | ||||
ΦK | −3.7576 | ||||
ϕJ | −0.0229 | ||||
ϕJK | −0.2262 | ||||
ϕK | 0.7187 | ||||
a-CH332SCH2f | |||||
Parameter | Calculated | Scaled | Experiment Hayashi et al. (1989) | ||
CCSDc | CCSD(T)d | CCSDe | |||
A0 | 16578.647 | 16572.974 | 16557.528 | 16556.964(25) | |
B0 | 7344.446 | 7342.763 | 7334.765 | 7335.312(5) | |
C0 | 5519.346 | 5518.023 | 5511.869 | 5512.033(6) | |
ΔJ | 8.124 | 8.404 | 7.865 | 8.02(11) | |
ΔJK | −34.249 | −35.179 | −31.683 | −32.27(116) | |
ΔK | 113.432 | 114.459 | 114.358 | 110.73(501) | |
δJ | 2.917 | 3.031 | 2.771 | 2.78(7) | |
δK | 2.267 | 2.291 | 2.567 | 2.35(138) | |
ΦJ | −0.0610 | ||||
ΦJK | 0.0569 | ||||
ΦKJ | 1.1229 | ||||
ΦK | −3.3337 | ||||
ϕJ | −0.0304 | ||||
ϕJK | −0.2534 | ||||
ϕK | 0.5996 | ||||
s-CH332SCH2Dg | |||||
Parameter | Calculated | Scaled | Experiment Hayashi et al. (1989) | ||
CCSDc | CCSD(T)d | CCSDe | |||
A0 | 17819.084 | 17812.372 | 17796.684 | 17795.061(38) | |
B0 | 7077.495 | 7076.097 | 7068.186 | 7068.973(10) | |
C0 | 5408.647 | 5377.454 | 5401.313 | 5401.701(9) | |
ΔJ | 6.504 | 6.721 | 6.297 | 6.87(21) | |
ΔJK | −31.194 | −31.985 | −31.448 | −27.50(85) | |
ΔK | 133.574 | 134.645 | 123.569 | 134.88(850) | |
δJ | 2.234 | 2.321 | 2.123 | 2.02 | |
δK | 3.475 | 3.540 | 3.935 | ||
ΦJ | −0.0480 | ||||
ΦJK | −0.1374 | ||||
ΦKJ | 2.8026 | ||||
ΦK | −8.7476 | ||||
ϕJ | −0.0239 | ||||
ϕJK | −0.3493 | ||||
ϕK | 0.6581 |
Notes. aRotational constants in MHz; quartic centrifugal-distortion constants in kHz; sextic centrifugal-distortion constants in Hz. Watson A-reduction in the I r representation is used. bEquilibrium constants corresponding to the best-estimated equilibrium structure (see text; Senent et al. 2014). Vibrational corrections to rotational constants, and quartic and sextic centrifugal-distortion constants at the MP2/cc-pVTZ level. cEquilibrium constants corresponding to the best-estimated equilibrium structure (see text; Senent et al. 2014). Vibrational corrections to rotational constants, and quartic and sextic centrifugal-distortion constants at the CCSD/cc-pVTZ level. dEquilibrium constants corresponding to the best-estimated equilibrium structure (see text; Senent et al. 2014). Vibrational corrections to rotational constants, and quartic and sextic centrifugal-distortion constants at the CCSD(T)/cc-pVTZ level. eEmpirically scaled parameters (see text) starting from those computed as at footnote c. fDeuterium substitution leads to a complete symmetry loss: from the C2V symmetry point group to C1. gDeuterium substitution leads to a limited symmetry loss: from C2V to CS.
Based on comparison with experiments, we note very good agreement between the latter and our best data. Indeed, we note mean discrepancies of about 0.12% and 0.07% when the best-estimated equilibrium constants (from the best-estimated equilibrium structures computed in Senent et al. 2014) are corrected for vibrational corrections at the CCSD/cc-pVTZ and CCSD(T)/cc-pVTZ levels, respectively. Employing MP2/cc-pVTZ corrections does not worsen the agreement, with mean discrepancies still on the order of 0.12%. Moving to quartic centrifugal-distortion constants, the MP2, CCSD, and CCSD(T) levels in conjunction with the cc-pVTZ basis set show averaged discrepancies of about 6.4%, 5.0%, and 6.1%, respectively. Sextic centrifugal-distortion constants deserve special note since, to our knowledge, only a few experimental values are available for the main isotopologue of DMS (Vacherand et al. 1987, see Table 1) and for the 32S- and 34S-containing isotopologues of gauche-ETSH and for 32S-trans-ETSH. While for DMS only a limited number of sextics are experimentally known, and therefore the poor agreement can be ascribed to limitations in the experimental work, for both gauche-and trans-ETSH the full sets of sextic centrifugal-distortion constants were determined in conjunction with higher-order terms. Therefore, a thorough inspection of this disagreement is required, especially because a sign inversion is observed in almost all cases. The corresponding discussion is reported later in the text.
Based on the results of Tables 1–3, we also note improved accuracy once we apply the empirical scaling procedure described above. In fact, the discrepancies decrease to less than 0.01% for rotational constants and to 3.1% (<2.0% in most cases) on average for quartic centrifugal-distortion terms. This improvement also has repercussions for the prediction of rotational transitions. In fact, our best-estimated computed parameters provide predictions with a relative accuracy of ∼0.1% in the centimeter/millimeter-wave region. Therefore, a rotational frequency of 200 GHz is currently predicted with an accuracy of 200 MHz. For the same frequency, the relative error decreases to ∼0.01%, resulting in an accuracy of 20 MHz for our prediction of a rotational transition at 200 GHz.
Our overall conclusion is that our predicted parameters, also the nonscaled ones, are reliable. We thus consider them to be sufficiently accurate for supporting laboratory or astronomical assignments and identifications. From the discussion above and from a methodological point of view, we would like to point out that the MP2/cc-pVTZ level is suitable for obtaining vibrational corrections to rotational constants and centrifugal-distortion parameters with an accuracy that allows for quantitative predictions.
Concerning the sextic centrifugal-distortion constants of gauche- and trans-ETSH, as mentioned above, an essentially complete disagreement is observed. For the trans form, the experimental parameters are affected by large uncertainties and we also note that the quartic term ΔK is enlarged as it moves from gauche to trans, while theory predicts the opposite trend. In the case of gauche-ETSH, however, the sextics are well determined. The sextics are very similar to our computed values but are opposite in sign. Based on the available literature for these topics (see, for example, Puzzarini et al. 2012a, 2012b, 2014b), sextic centrifugal-distortion constants computed at the CCSD(T)/cc-pVTZ level usually have an averaged accuracy of about 10% and show maximum discrepancies of about 20%. Therefore, we suggest that we further investigate the rotational spectra of gauche- and trans-ETSH.
3.2. Torsional Energy Levels and Splittings
Table 4 summarizes the lowest torsional energy levels (ground and first torsional states) corresponding to the various isotopic species of g-ETSH, t-ETSH, and DMS. Second torsional states, combination bands, and vibrational partition functions are also provided in our tables. The levels are classified using the representations of the G6 (ETSH) and G36 (DMS) Molecular Symmetry Groups and using two vibrational quanta. For ETSH, ν20 and ν21 refer to the methyl torsion and the hydroxyl torsion, respectively. For DMS, ν15 represents the infrared active mode. For both g-ETSH and t-ETSH, the vibrational ground state A/E splitting caused by the methyl internal rotation is very small. Nevertheless, the vibrational ground state of the hydrogenated isotopologues of g-ETSH splits by ∼0.060 cm−1 as a consequence of the SH torsion; this splitting largely reduces and becomes very small (only 0.002 cm−1) in the deuterated species. The vibrational ground state of DMS splits into nine components. The levy of degeneracy leads to energy separations of less than 0.001 cm−1. This means that the rotational study of the vibrational ground state of DMS using a treatment for semi-rigid molecules is realistic. For the first and second excited states, non-rigidity needs to be considered.
Table 4. Low Torsional Energy Levels (in cm−1) of Ethyl Mercaptan and Dimethyl Sulfide Isotopologues Calculated at the CCSD(T)/aug-cc-pVTZ Level
gauche- ethyl-mercaptan | ||||||
---|---|---|---|---|---|---|
CH3CH232SH | CH3CH234SH | CH3CH236SH | CH3CH233SH | CH3CH232SD | ||
ZPVE | 226.898 | 226.773 | 226.661 | 226.834 | 201.658 | |
ν20, ν21 | ||||||
0 0+ | A1, E | 0.000 | 0.000 | 0.000 | 0.000 | 0.000 |
0 0− | A2, E | 0.061 | 0.060 | 0.059 | 0.060 | 0.002 |
0 1+ | A1, E | 188.132 | 188.086 | 188.043 | 188.108 | 145.434 |
0 1− | A2, E | 189.613 | 189.552 | 189.497 | 189.582 | 145.525 |
1 0+ | A1 | 254.046 | 253.888 | 253.745 | 253.965 | 252.600 |
E | 254.045 | 253.887 | 253.746 | 253.964 | 252.600 | |
1 0− | A2 | 254.068 | 253.910 | 253.769 | 253.986 | 252.599 |
E | 254.067 | 253.909 | 253.768 | 253.985 | 252.598 | |
0 2+ | A1, E | 339.848 | 339.824 | 339.802 | 339.835 | 275.486 |
0 2− | A2, E | 356.237 | 356.102 | 355.981 | 356.168 | 276.729 |
1 1+ | A1 | 441.675 | 441.493 | 441.331 | 441.581 | 398.580 |
E | 441.677 | 491.496 | 441.334 | 441.584 | 398.581 | |
1 1− | A2 | 441.334 | 441.175 | 441.033 | 441.252 | 398.982 |
E | 441.336 | 441.178 | 441.036 | 441.255 | 398.983 | |
2 0+ | A1 | 491.359 | 491.049 | 490.772 | 491.199 | 487.356 |
E | 491.145 | 491.074 | 490.797 | 491.223 | 487.369 | |
2 0− | A2 | 491.120 | 490.804 | 490.521 | 490.957 | 487.343 |
E | 491.384 | 490.829 | 490.546 | 490.982 | 487.383 | |
trans- ethyl-mercaptan | ||||||
CH3CH232SH | CH3CH234SH | CH3CH236SH | CH3CH233SH | CH3CH232SD | ||
ν20, ν21 | ||||||
0 0 | A1, E | 157.835 | 157.991 | 158.133 | 157.915 | 160.083 |
0 1 | A2, E | 313.572 | 313.669 | 313.760 | 313.622 | 283.577 |
1 0 | A2 | 403.069 | 403.146 | 403.218 | 403.108 | 403.069 |
E | 403.067 | 403.145 | 403.217 | 403.107 | 403.068 | |
0 2 | A1, E | 424.286 | 424.349 | 424.409 | 424.318 | 392.125 |
1 1 | A1 | 555.804 | 555.706 | 555.622 | 555.753 | 522.955 |
E | 555.807 | 555.709 | 555.624 | 555.756 | 522.956 | |
2 0 | A1 | 632.303 | 632.332 | 632.362 | 632.317 | 628.722 |
E | 632.342 | 632.371 | 632.400 | 632.357 | 628.765 | |
Dimethyl sulfide | ||||||
CH332SCH3 | CH334SCH3 | CH336SCH3 | CH333SCH3 | |||
ZPVE | 187.066 | 186.905 | 186.761 | 186.983 | ||
ν11, ν15 | ||||||
0 0 | A1 | 0.000 | 0.000 | 0.000 | 0.000 | |
G, E1, E3 | 0.001 | 0.001 | 0.001 | 0.001 | ||
1 0 | A3 | 176.516 | 176.530 | 176.542 | 176.523 | |
G | 176.500 | 176.514 | 176.526 | 176.507 | ||
E2, E3 | 176.484 | 176.498 | 176.511 | 176.491 | ||
0 1 | A2 | 182.289 | 181.983 | 181.709 | 182.131 | |
G | 182.273 | 181.967 | 181.694 | 182.116 | ||
E1, E4 | 182.258 | 181.952 | 181.678 | 182.100 | ||
2 0 | A1 | 339.979 | 339.888 | 339.802 | 339.933 | |
G | 340.213 | 340.114 | 340.018 | 340.163 | ||
E1, E3 | 340.587 | 340.494 | 340.406 | 340.540 | ||
1 1 | A4 | 341.312 | 341.058 | 340.831 | 341.181 | |
G | 341.712 | 341.462 | 341.240 | 341.583 | ||
E2, E4 | 341.972 | 341.711 | 341.478 | 341.837 | ||
0 2 | A1 | 361.048 | 360.590 | 360.185 | 360.811 | |
G | 361.044 | 360.584 | 360.177 | 360.806 | ||
E1, E3 | 361.040 | 360.577 | 360.169 | 360.800 |
Download table as: ASCIITypeset image
The variation of the torsional energies with the substitution of 32S for other sulfur isotopes is very small (less than 1 cm−1) because only the hydrogen atoms are significantly displaced during the internal rotation. Nevertheless, the variation is very important for the SH→SD substitution. For example, the OH fundamental levels vary from 188.132 cm−1 and 189.613 cm−1 to 145.434 cm−1 and 145.525 cm−1. The CH3 fundamentals vary from 254.046 (5) cm−1 and 254.068(7) cm−1 to 252.600 cm−1 and 252.599(8) cm−1. It must be considered that deuterium is a cosmological abundant isotope. CH3CH2SD represent the most probable detectable compound.
4. CONCLUDING REMARKS
The present contribution provides an accurate and reliable set of spectroscopic parameters for different isotopic species of DMS and ETSH. For this purpose, state-of-the-art computational methods and approaches have been employed. We thus determined highly accurate rotational and torsional parameters. Available experimental data were used to assess the reliability of our computations. For instance, relative accuracies of 0.1% and 5%–6% were observed for rotational and quartic centrifugal-distortion constants, respectively, and were further improved through empirical scaling. The rotational-spectroscopy characterization was complemented by the calculation of reliable sextic centrifugal-distortion terms. On the whole, our computed parameters allow us to predict rotational transitions with the proper accuracy for future laboratory and/or astronomical investigations.
The A/E splitting of the ground vibrational state of ETSH caused by the A/E torsion is almost negligible. However, a splitting of ∼0.060 cm−1 due to the SH torsion is observed for the hydrogenated isotopologues of g-ETSH; this splitting is negligible in the deuterated species. In DMS, the low-energy torsional states split into nine components with separations of less than 0.001 cm−1. Therefore, the vibrational ground-state rotational study based on the semi-rigid rotor approximation should be considered reliable; meanwhile, an appropriate treatment accounting for non-rigidity is required to describe the first and second excited states.
The overall conclusion is that based on the good agreement and well-established computational techniques employed, we are confident that the spectroscopic data provided herein are highly accurate and can therefore be useful for the identification of rare isotopologues of DMS and ETSH in the interstellar medium.
This research was supported by a Marie Curie International Research Staff Exchange Scheme Fellowship within the 7th European Community Framework Program under grant No. PIRSES-GA-2012‐31754, the COST Action CM1002 CODECS, and the FIS2011‐28738-C02‐02 project (MINECO, Spain). In Bologna, this work was supported by MIUR (PRIN 2012 funds under the project "STAR: Spectroscopic and computational Techniques for Astrophysical and atmospheric Research") and by the University of Bologna (RFO funds). M.L.S., M.H., and M.A.M. acknowledge the Deanship of Scientific Research at King Saud University for its funding through the Research Group RGP-VPP-333.
The authors acknowledge CTI (CSIC) and CESGA for computing facilities.