Abstract
Particle-in-cell simulations have been conducted to investigate the parametric decay processes of radiofrequency waves in the ion cyclotron range of frequency in inhomogeneous plasmas. By choosing the parameters close to those in the ion Bernstein wave heating experiments on the HT-7 tokamak (Li et al 2001 Plasma Phys. Control. Fusion 43 1227–38), decay channels similar to experimental observations have been found in simulations. It is shown that due to strong inhomogeneity of tokamak edge plasma, layers may exist where selection rules of the mode–mode coupling are satisfied, and resonant parametric decays can occur if the input wave power is sufficiently high. As a result, daughter waves at half-, second- and third-harmonic frequencies of the pump wave can be excited. The nonresonant parametric decay is also observed, and ions are heated via nonlinear Landau damping.
Export citation and abstract BibTeX RIS
1. Introduction
Parametric decay instability (PDI), one of the nonlinear interactions between radiofrequency (rf) waves and plasma, has attracted considerable attention in the last several decades [1–5]. PDI describes a three-wave coupling process where a pump wave with frequency ω0 and wavenumber k0 decays into two daughter waves with frequencies ω1, ω2 and wavenumbers k1, k2 when the selection rules ω0 = ω1 + ω2, k0 = k1 + k2 are satisfied. The PDI process can be categorized into two types [1, 2]: the resonant mode–mode coupling in which all the daughter waves are propagating waves, and the nonresonant mode–mode coupling (or nonlinear Landau damping) where at least one of the daughter waves is a quasimode, which will be heavily damped on electrons or ions.
In a homogeneous plasma, the theories of PDI were developed during the 1960s and 1970s [1]. In order to test these theories, experiments were carried out for both resonant and nonresonant decay processes. In these experiments, the frequency and wavenumber selection rules as well as the elements of a nonlinear coupling matrix were measured quantitatively and found to be in agreement with the theory within experimental error [1, 6, 7]. In an inhomogeneous plasma, the wavenumber-matching conditions for mode–mode coupling are only satisfied locally. As the waves move away from this region, the matching of the selection rules is destroyed and a higher threshold of instability is required due to the convective loss [1, 2]. With a WKB-type phase approximation [8], the convective amplification factor and thus the threshold pump power of resonant three wave parametric instability are obtained in a weakly inhomogeneous plasma [1, 2]. In addition, finite extent or nonuniform pumps also limit the size of the interaction region and introduce convection losses [1, 2]. While the PDI theory in uniform or weakly inhomogeneous plasmas is well understood, the understanding of this phenomenon in strongly inhomogeneous plasmas, such as tokamak plasmas, is rather poor.
With more and more applications of rf waves for plasma heating and current drive on tokamak devices, the interactions between waves and plasmas have been investigated extensively [9–18]. When the input power of the incident wave reaches a megawatt, nonlinear phenomena occur and cannot be ignored. The observations of PDI during the ion cyclotron range of frequency (ICRF) heating experiments have been reported on different tokamak devices, such as ASDEX [10], JET [11], TEXTOR [12, 13], JT-60 [14], DIII-D [9, 15, 16], HT-7 [17], Alcator C-Mod [18], etc. In these experiments, the observed decay processes involved (1) resonant decay into two ion Bernstein waves (IBWs), (2) nonresonant decay into an ion cyclotron quasimode (ICQM) plus an IBW, and (3) nonresonant decay into a low-frequency electron quasimode (EQM) and an IBW. The frequency spectra of these decay processes were well measured. However, the structures of these observed waves, specifically their amplitudes, wavelengths and spatial extent, were not measured in the experiments due to the shortage of the diagnostics. Thus the experimental observations were not well explained and the PDI mechanism was not fully understood.
Numerical simulation provides a tool for better understanding of the existing experiments. Different simulation codes based on WKB and full wave descriptions of plasma–wave interactions have been developed [19–21]. Unfortunately, these codes are applicable mainly in the linear regime and thus cannot capture the nonlinear physics. Particle-in-cell (PIC) approach is a good candidate for nonlinear simulations. However, there are only a few numerical studies that focus on the excitation and propagation of rf waves in a magnetic fusion-relevant plasma using PIC codes. In the linear regime, the excitation and propagation of IBWs have been investigated with an electrostatic 2D PIC code [22] and in cylindrical geometry of the gyrokinetic toroidal code (GTC) [23]. In the nonlinear regime, simulations of the PDI processes include decays of an electron Bernstein wave [24, 25] and IBW [26]. In [26], the computational calculation provided a similar frequency spectrum with the IBW experiment on Alcator C-Mod [18] when the plasma parameters were chosen to match. In this reference, the authors focused on the capability of fluid and PIC models to capture the PDI simulation rather than the mechanism of the PDI process.
In this paper, the PIC method has been used to investigate the collisionless nonlinear interactions between plasmas and ICRF, aiming to better understand the mechanism of the PDI process and interpret the relevant experiments. We consider an inhomogeneous plasma with both density and magnetic field varying with the radial position. The simulation parameters have been chosen to resemble those of the IBW experiments on the HT-7 tokamak [17]. In the simulations, we obtain resonant and nonresonant parametric decay channels similar to those observed in the experiments. From the simulations, not only are the frequency-matching conditions checked, but also the spatial distributions of the electric field of the waves are given, thus the wavenumber-matching conditions can be shown. It is demonstrated that the resonant decay is excited only at the place where the matching conditions are satisfied, and the nonresonant decay is most likely triggered in the vicinity of the cold plasma resonance layer where the electric field |E| of the pump wave reaches its maximum value.
The rest of this paper is organized as follows. Section 2 describes the simulation model and its verification in the linear regime with a low input power. As the input power increase, the resonant and nonresonant decay processes are investigated and presented in section 3. Finally, the paper is concluded with a summary and discussion in section 4.
2. Simulation model and verification in the linear regime
In the present work, simulations via the full PIC method in the VORPAL framework [27] have been taken to study the PDI process of ICRF in the edge region of tokamak plasma. Since PDI spectra have been well measured during IBW heating experiments on the HT-7 tokamak [17], we try to give an interpretation of the generation of these modes here. Thus we take simulation parameters as close as possible to those used in HT-7 experiments. In this section, we will introduce our simulation model and verify it in the linear regime.
2.1. Simulation model
The simulation model is shown in figure 1. We consider a slab model of tokamak plasma with the external magnetic field along the y (toroidal) direction, and the magnetic field and density gradient in the x (radial) direction. The z direction denotes the poloidal direction. The simulation length in the x direction is lx = 0.06 m. There is a vacuum region between the left boundary x = 0 m and the plasma edge x0 = 0.0012 m. Since the plasma density profile is unavailable in the experiments, we assume it to be n(x) = n0[0.43a tan (5x/lx − 2) + 0.47], as shown in figure 1, where n0 = 2.0 × 1017 m−3. A perturbation current is placed in the vacuum with the expression J(x, t) = J0exp(−(x − 0.0006)2/0.00062) sin (ω0t)(1−exp(−γt))2, where γ = 0.02ω0 and (1 − exp(−γt))2 is a ramped factor used to avoid the excitation of the local modes [28]. The thin vacuum layer on the left edge is used to reduce the effect of particle perturbation on this current source. A pump wave with frequency ω0 is launched and then propagates into the plasma. In the simulation, 900 particles per cell are typically used for the perturbation current amplitude J0 = 2 × 102 A m−2. These particles are loaded as the Maxwellian distribution in velocity space and uniformly in space. When the particles reach the left or right boundary, they are absorbed and removed from the simulation. Both electrons and ions are treated by a fully kinetic model. For simplicity, the plasma temperature is assumed to be uniform in the simulation region. The temperatures of the ions and electrons are 30 eV and 50 eV, respectively. Typically, the spatial grid resolution is Δx/ρi ≃ 0.08, which is high enough to resolve the ion gyro motion. The typical duration of our simulations is several hundred of rf periods, which brings us the high frequency resolution during the FFT and takes approximately 24 h with 64 cores on our cluster.
Figure 1. Simulation model and the plasma density profile.
Download figure:
Standard image High-resolution image2.2. Verification of the simulation model
From the linear theory, the launched electron plasma wave (EPW) propagates towards the cold plasma resonance layer and then transforms smoothly into the IBW in an inhomogeneous plasma, arising from the finite ion-Larmor-radius effect [9, 29]. The relevant electrostatic dispersion relation can be expressed as [9]

where Kxx,Kxy and Kzz are elements of the plasma dielectric tensor and can be written as
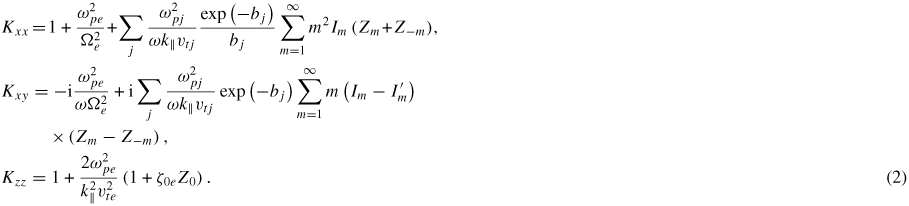
In equation (2), the summation j is over ion species, m is the harmonic number, Zm is the plasma dispersion function with argument ζmα ≡ (ω + mΩα)/k∥vtα,
, α denotes species, and Im is the modified Bessel function with argument
. The cold plasma resonance, which separates EPW and IBW regions, occurs when Kxx vanishes in the limit Ti = 0.
In order to reproduce this mode-transformation process, a plasma with single ion species (hydrogen) is taken into account. The density profile is kept the same as shown in figure 1 and the external magnetic field is uniform and B0 = 1.2T. The injected frequency ω0 = 1.77ΩH. The ion gyroradius of hydrogen ρH = vtH/ΩH = 6.6 × 10−4 m and k⊥ρH ≃ 0.8 at x = 0.02 m. With these parameters, the corresponding dispersion curve, i.e. the perpendicular wavenumber versus the radial position, is plotted as the blue solid line in figure 2(a). The cold plasma resonance layer locates at x = 0.009 m. The spatial distribution of Ex for the driving frequency from the simulation is illustrated in figure 2(b). It shows that the launched EPW transforms to an IBW and then penetrates into the plasma interior. The perpendicular wavenumber k⊥ calculated from the simulation is compared with the linear dispersion curve, and excellent agreement is shown in figure 2(a). The frequency spectrum of the wave amplitude |Ex| in figure 2(c) indicates that only the peak of the driving frequency appears, and no sideband is present.
Figure 2. (a) Dispersion curve for the injected wave (blue solid line) and the measured values of k⊥ from the simulation (red circles). (b) Spatial distributions of the electric field (Ex) of the injected wave. (c) The frequency spectrum of Ex at x = 0.02 m and t = 487/ω0 with a low input power.
Download figure:
Standard image High-resolution imageFor a low incident rf power, our simulation provides the mode converted electrostatic IBW as predicted by the linear theory. Good agreement in the comparison justifies our simulation model.
3. Observation of PDI processes
To study the nonlinear behaviour of rf waves propagating in plasma, we increase the input power of the pump wave in our simulations. Both resonant and nonresonant PDI processes are observed. Before showing our simulation results, we first give a brief review of the observations of PDI during IBW heating experiments on the HT-7 tokamak [17]. In the frequency spectra of these experiments, the detected peaks included (1) the second and third harmonics of the pump wave, (2) harmonics of the half-pump frequency, i.e.
and
, and (3) two nonresonant decay pairs, i.e. ICQM+IBW and low-frequency EQM + IBW. To reduce our computational cost, we choose a low k∥ to exclude the electron contributions to the PDI process. Since the driving frequency satisfies ω0 ≫ k∥vte in our simulations, the process of decaying into an IBW plus an EQM does not appear. The other PDI processes are investigated and presented below.
3.1. Excitation of resonant mode–mode coupling
To investigate the PDI process, we raise the perturbation current amplitude to J0 = 6 × 102 A m−2 and the obtained electric field is about several 104 V m−1, which is close to that observed in the experiment. While other parameters are kept the same as in the above case, the second harmonic of the pump wave is observed in the simulation. The spatial distributions of Ex for the pump and second harmonic waves at different times are shown in figure 3. Clearly, the second harmonic wave is generated around the place x = 0.02 m.
Figure 3. Spatial distributions of the electric fields with the injected frequency (a), (c), (e) and its second harmonic (b), (d), (f) at t = 142/ω0 (top), t = 203/ω0 (middle) and t = 264/ω0 (bottom).
Download figure:
Standard image High-resolution imageIn order to explain the generation of the second harmonic wave, we plot the linear dispersion curves of the injected frequency and its second harmonic in figure 4 as the blue solid line and black dashed–dotted line, respectively. It is shown that the selection rule
for the resonant mode–mode coupling can be satisfied at x ≃ 0.02 m (indicated as the vertical magenta dashed–dotted line). This suggests that the second harmonic wave could be resonantly excited. The wavenumbers of the pump wave and its second harmonic are compared with the linear dispersion relation of IBWs in figure 4. Good agreement in the comparison shows that the second harmonic wave is an IBW generated by the resonant PDI process.
Figure 4. Dispersion curves k⊥ versus x for the pump wave (blue solid line), second harmonic (black dot line), third harmonic (red crosses) and half-harmonic (light green dots). The vertical magenta dashed–dotted and cyan dashed lines denote the radial positions x = 0.02 m and x = 0.026 m, respectively. Magenta circles are the measured values of k⊥(ω0) and green triangles are of k⊥(2ω0) from the simulation.
Download figure:
Standard image High-resolution imageIt is also noticed that the selection rule
can be matched at x ≃ 0.026 m (indicated as the vertical cyan dash line in figure 4). One would expect the third harmonic wave to be excited if the amplitudes of the pump and the second harmonic waves are large enough. Such three-wave interaction is indeed observed in the simulation. Figure 5 illustrates the contours of the amplitude of |E| in the x − ω plane at different times from the simulation. In figure 5(a), one can see that the pump wave is generated and propagates into the plasma. As time goes on, the second harmonic wave appears around x = 0.02 m (indicated by the vertical magenta dashed–dotted line) where
and its amplitude increases (figures 5(b) and (c)). As shown in figure 4, the group velocity of the second harmonic wave is very small near the resonant region. Thus the second harmonic wave propagates slowly in this region. Later on, as both the pump and second harmonic waves pass through the position x = 0.026 m (indicated by the vertical cyan dashed line), where
, a weak third harmonic wave is excited (figure 5(d)) and then propagates into the plasma (figures 5(e) and (f)).
Figure 5. Contours of the amplitude of |E| at different times: (a) t = 81/ω0, (b) t = 142/ω0, (c) t = 203/ω0, (d) t = 264/ω0, (e) t = 325/ω0 and (f) t = 386/ω0. The vertical magenta dashed-dotted and cyan dashed lines denote the radial positions x = 0.02 m and x = 0.026 m, respectively.
Download figure:
Standard image High-resolution imageA comparison of the frequency spectrum between our simulation result and the experimental observation is displayed in figure 6. One type of wave spectrum measured on the HT-7 tokamak during an IBW heating experiment [17], illustrated in figure 6(a), shows that the peaks of the half (fD), second (f2H) and third (f3H) harmonics of the pump (f0) were observed. In the wave spectrum obtained from the simulation (figure 6(b)), the waves with the frequencies of the pump (ω0), second (2ω0) and third (3ω0) harmonics are also found. The half-pump frequency is absent because the simulation parameters (for instance, the density and temperature profiles) are not exactly the same as those in the experiment. In the simulation, the matching condition
is satisfied at the place x = 0.008 m where the amplitude of the pump wave is too small to drive the nonlinear PDI process. The simulation result indicates that the generation of the second harmonic mode is due to the resonant self-interaction of the incident wave as discussed in [24], while the excitation of the third harmonic mode is from the resonant mode–mode coupling between the pump wave and the generated second harmonic wave. Meanwhile, the zero-frequency mode
is also produced in figure 6(b), which was also observed in [30].
Figure 6. (a) One type of wave spectrum in the HT-7 experiment, the peaks of half (fD), second (f2H) and third (f3H) harmonics of the pump (f0) were observed (reprinted with permission from [17], Copyright 2001 IOP Publishing). (b) The frequency spectrum at x = 0.024 m and t = 487/ω0 in the simulation.
Download figure:
Standard image High-resolution imageIn the above case, the harmonics of the half-pump frequency wave do not appear in our simulation, while they have been observed in the experiments. In order to explore the generation of these modes, we change some of the plasma parameters to ensure the relevant selection rules can be satisfied in the simulation region. We consider a deuterium plasma with hydrogen minority and set the hydrogen concentration H/(H+D) = 0.15. The density profile is n(x) = n0[(x − x0)2/lx2] with n0 = 2.5 × 1017 m−3 and the magnetic field varies along the x direction with the form B(x) = 1.57/(1.26 − x) T. The injected frequency is in the range of ω0 = (1.70 ∼ 1.62)ΩH and the ratio ω0/ΩH = 1.67 at x = 0.023 m.
The dispersion curves of this case for the driving frequency ω0 and its half-harmonics 0.5ω0 and 1.5ω0 are plotted in figure 7. It shows that the selection conditions
and
are satisfied around x ≃ 0.023 m. It is implied that the waves with frequencies 0.5ω0 and 1.5ω0 could be produced by the resonant parametric decay if the amplitude of the pump wave is large enough.
Figure 7. Dispersion curves k⊥(x, ω0) (blue solid line), k⊥(x, 0.5ω0) (black dot line) and k⊥(x, 1.5ω0) (red crosses) vary with x. The magenta dashed-dotted and cyan dashed lines denote the values 2k⊥(x, 0.5ω0) and k⊥(x, 0.5ω0) + k⊥(x, ω0), respectively. Green triangles are the measured values of k⊥(0.5ω0) from the simulation. The only cyclotron resonance that appears in this case is 1.5ω0 = 5ΩD, which is marked as the vertical red arrow.
Download figure:
Standard image High-resolution imageFigure 8 illuminates the history of the spatial variation of Ex for the pump wave and its half-harmonic. It is demonstrated that initially the half-pump frequency wave is present in the vicinity of the resonance layer. Later in time, the half-pump frequency wave grows and propagates into the plasma. The wavenumber of the half-pump frequency wave calculated from the simulation is dotted as the green triangles in the dispersion relation shown in figure 7, and it agrees well with the linear theory. Good agreement in the comparison confirms that the half-pump frequency wave is an IBW generated by the resonant PDI process.
Figure 8. The spatial variation of Ex for the pump wave (a), (c) and the half-pump frequency (b), (d) at t = 203/ω0 (top) and t = 487/ω0 (bottom).
Download figure:
Standard image High-resolution imageFigure 9 shows the comparison of the wave spectrum between the simulation and the experiment on the HT-7 tokamak [17]. In figure 9(a), a typical wave spectrum of PDI during IBW heating experiment on HT-7 [17] is illustrated. Obviously, the second harmonics (f2H) and the harmonics of the half-pump frequency, including 0.5f0, 1.5f0 and 2.5f0 (indicated as fD, f3D and f5D), were detected in the experiment. The frequency spectrum of |Ex|2in figure 9(b) indicates that waves with the frequency of ω0, 0.5ω0, 1.5ω0 and 2ω0 are also generated in our simulation. Similarly, the wave with frequency 2.5ω0 does not appear due to the mismatch of the k-matching condition. Our simulation result suggests that the half-harmonics of the pump wave are generated by the resonant mode–mode coupling. These resonant parametric decays are observed near the layers where the selection rules of mode–mode coupling are satisfied in inhomogeneous plasmas. If the selection rules are not satisfied, the corresponding waves are not observed in the simulations.
Figure 9. (a) A typical wave spectrum of PDI in an HT-7 experiment (reprinted with permission from [17], Copyright 2001 IOP Publishing). Waves with frequencies 0.5f0, 1.5f0, 2.5f0 (indicated as fD, f3D, f5D) and 2f0 (indicated as f2H) were observed in the experiment. (b) The frequency spectrum at x = 0.0274 m and t = 487/ω0 in the simulation.
Download figure:
Standard image High-resolution image3.2. Generation of nonresonant mode–mode coupling (nonlinear Landau damping)
In addition to the resonant parametric decay described in the previous subsection, nonresonant decay processes were also observed in the IBW heating experiments on the HT-7 tokamak [17]. To show these nonresonant PDI processes, we choose plasma parameters that remove all the resonant decay channels depicted above. The plasma density profile is kept the same as in the half-harmonics case while we set n0 = 2.0 × 1017 m−3. The magnetic field is raised up a bit as B(x) = 1.93/(1.53 − x) T. The injected frequency is ω0 ≈ 1.25ΩH. The hydrogen concentration is set to H/(H + D) = 0.2 in this case. From the linear dispersion curves, second and third harmonics and half-harmonics frequencies cannot be resonantly generated in this case. We focus on the nonresonant mode–mode coupling in this subsection.
The time evolution of the contours of |E| in the x − ω plane (top) and normalized kinetic energy of deuterium (bottom) in the simulation are shown in figure 10. Figure 10(b) illustrates that the ICQM of D with frequency ΩD ≃ 0.4ω0 (marked as the white dashed–dotted horizontal line) and the corresponding beat wave with frequency 0.6ω0 ≃ 1 − ΩD (marked as the white dashed horizontal line) are excited near the cold plasma resonance layer at t = 397/ω0. Simultaneously, the ion heating is significant at those places (figure 10(d)). However, ICQMs do not appear and no ion heating is observed in the resonant decay processes discussed above. Thus we believe that the ion heating might be caused by the heavily damping ICQMs produced by the nonresonant PDI process. Obviously, the energy of the pump wave will strongly deposit at the plasma edge due to the nonlinear Landau damping.
Figure 10. Contours of |E| in the x − ω plane at (a) t = 72/ω0 and (b) t = 397/ω0. The normalized kinetic energy of deuterium at (c) t = 72/ω0 and (d) t = 397/ω0.
Download figure:
Standard image High-resolution imageIn the above case, we show that only one nonresonant decay channel appears in the simulation. However, different decay channels may be excited simultaneously as observed in the experiments. For given plasma parameters, decay channels with the growth rate exceeding its damping rate will grow and compete with each other. Figure 11 illuminates an example that several decay channels coexist in the same time. In this case, the density profile is n(x) = n0[0.37a tan (10x/lx − 4) + 0.49] with n0 = 7.5 × 1016 m−3 and the other parameters are kept the same as the half-harmonics case. The selection rule
can be matched in this case. The figure shows that the peaks of 0.4ω0 = ω0 −ΩH (the beat wave between the injected wave and ICQM of H), 0.5ω0 and 0.7ω0 = ω0 − ΩD (the beat wave between the injected wave and ICQM of D) are observed. The corresponding ICQMs (ΩH = 0.6ω0 and ΩD = 0.3ω0) may be heavily damped on the ions and are absent in the frequency spectrum. Both H and D ions heating are found in this case.
Figure 11. An example of PDI spectrum shows the coexistence of different parametric decay channels in the simulation.
Download figure:
Standard image High-resolution image4. Summary and discussion
In this work, PIC simulations are performed to study the parametric decay processes of rf waves in the ion cyclotron range of frequency in inhomogeneous plasmas.
We first verify our simulation model by comparing the simulation results with linear theory. When the input power of the incident wave is low, the simulation reproduces the mode transform process from an injected electron plasma wave to an ion Bernstein wave, which is well predicted by the linear theory. Good agreement in the comparison justifies our simulation model.
With the input power increasing to a few hundreds of kilowatts, our simulations demonstrate similar parametric decay channels as those observed in the HT-7 IBW experiments [17]. It is suggested that the peaks of half-, second- and third-harmonic frequencies of the pump wave observed in the experiments on HT-7 [17] as well as TEXTOR [13] and DIII-D [15] might be generated by resonant PDI processes. In a tokamak plasma, inhomogeneity always exists especially near the plasma edge. In this region, as we have shown that kx varies very rapidly in space so that the matching condition for k may be readily satisfied. Therefore, the resonant decay processes are locally excited in the vicinity of the resonant layer. The daughter waves are not observed in the simulations when the corresponding selection rules are mismatched. In addition, the nonresonant mode–mode coupling is also detected in our simulations. In these nonresonant decay processes, the ion cyclotron quasimodes (mΩi) and the corresponding beat waves (ω0 − m Ωi) are generated and the ion heating is observed. The ion heating may be caused by the ion cyclotron resonant damping of the ICQMs and lead to the power absorption near the plasma edge, which may provide a mechanism to interpret the edge heating and ion tail formation observed in experiments [10, 12, 14, 18]. The nonresonant decay process is most likely triggered around the cold plasma resonance layer where the amplitude of the pump wave reaches its maximum value. Because most of the wave energy is exhausted here, PDI is not significant elsewhere. Obviously, the appearance of daughter waves may deposit wave energy at unexpected locations, such as the tokamak edge region rather than the plasma interior. As shown in the experiment as well as in our simulations, all the decay channels can be excited when the selection rules are satisfied. Competition exists among all of these different decay channels, no matter whether they are resonant or nonresonant coupling.
The validity condition for the theoretical work in weakly inhomogeneous plasmas is the usual WKB limit kL ≫ 1. In our simulations, we benchmark our results in the linear regime by comparing the wavenumber with the linear theory prediction, so we confined our discussions in the regime kL ≫ 1. We find that in this regime the simulation results are consistent with some theoretical predictions; for instance, PDI occurs in the place where the matching condition is locally satisfied, and the decay channels are as theoretically predicted. However, a more quantitative comparison should be made between the simulation results and theoretical predictions. For the next step, we are going to explore the regime kL ∼ 1 and find out how the plasma scale length affects PDI processes.
Acknowledgments
The authors would like to express their appreciation to Professor Li J and IOP Publishing for granting permission for the use of figures in the paper published as Plasma Phys. Control. Fusion 43 (2001) 1227–38. This work was supported by the JSPS-NRF-NSFC A3 Foresight Program in the field of Plasma Physics (NSFC: No. 11261140328 and NRF: No. 2012K2A2A6000443), the National Magnetic Confinement Fusion Science Program of China under Contract No. 2013GB111002, the National Natural Science Foundation of China under Contracts Nos 11475220 and 11475223 and by the Program of Fusion Reactor Physics and Digital Tokamak of the CAS 'One-Three-Five' Strategic Planning. Numerical computations were performed at the ShenMa High Performance Computing Cluster at the Institute of Plasma Physics, at the Chinese Academy of Sciences.