Abstract
Digital tomosynthesis (DT) is a limited angle tomographic x-ray technique. It is an attractive low-dose alternative to computed tomography (CT) in many imaging applications. However, the DT dataset is incomplete, which leads to out-of-focus artifacts and limited axial resolution. In this paper, a novel dual-axis tilt acquisition geometry is proposed and evaluated. This geometry solves some issues in tomosynthesis with the traditional scanning geometry by scanning the object with a set of perpendicular arcs. In this geometry the acquisition in the additional perpendicular direction is done using a tiltable object supporting platform. The proposed geometry allows for capturing more singularities of the Radon transform, filling the Fourier space with more data and better approximating the Tuy–Smith conditions. In order to evaluate the proposed system, several studies have been carried out. To validate the simulation setup the performance of the traditional scanning geometry has been simulated and compared to known results from the literature. It has also been shown that the possible improvement of the image quality in the traditional geometry is limited. These limitations can be partially overcome by using the proposed dual-axis tilt geometry. The novel geometry is superior and with the same number of projections better reconstructed images can be obtained. All studies have been made using a software tomosynthesis simulator. A micro-CT reconstruction of a bone has been used as a software phantom. Simultaneous algebraic reconstruction has been used to reconstruct simulated projections. As a conclusion, acquiring data outside the standard arc allows for improving performance of musculoskeletal tomosynthesis. With the proposed dual-axis acquisition geometry a performance gain is achieved without an increase in dose and major modifications to the instrumentation of existing tomosynthesis devices.
Export citation and abstract BibTeX RIS
1. Introduction
Digital tomosynthesis (DT) is a limited angle x-ray imaging technique. It is a non-invasive and non-destructive method for the three-dimensional visualization of the inner structures of an object. Tomosynthesis has a long history, starting when Grant in 1972 (Grant 1972) first proposed to use the word 'tomosynthesis'. The concept of tomosynthesis is based on the geometric tomography, which has been developed even earlier and can be found in literature e.g. in Ziedses des Plantes's (1932) work. Nowadays, DT regains scientific interest. It is known as an attractive low-dose alternative to computed tomography (CT) in medical and non-medical imaging applications, when the data over the full angular range is unavailable due to the properties of an object. DT is used, e.g. for the detection of microcalcifications and tumors in breast imaging (Niklason et al 1997), chest pulmonary nodules (e.g. Dobbins et al 2008, Tingberg 2010), in dental imaging (Ogawa et al 2010) or orthopedic applications (Duryea et al 2003). In this paper, DT is considered for the musculoskeletal imaging of hands.
The tomosynthesis projection dataset is incomplete in contrast to CT where projection data is acquired over 360° range. The incompleteness of the tomosynthesis dataset results in a missing wedge in Fourier space and lost singularities of the Radon transform (Quinto 1993). Thus, the Tuy–Smith sufficiency conditions (Tuy 1983, Smith 1985) are not fulfilled. This results in limited angle artifacts in reconstructed images. Although tomosynthesis is a volumetric imaging technique and provides dimensional information about the location of structures, the complete three-dimensional information about the object cannot be reconstructed. The resolution of the reconstructed tomosynthesis slices is anisotropic and the in-depth (axial) resolution is limited. Structures of interest, e.g. bone boundaries or joint margins in axial slices can hardly be delineated. They are blurred out or even completely lost. In-plane images are affected by out-of-focus artifacts. Features, which are located above and below the target plane appear in the plane of interest as multiple ghost copies. The intensity of out-of-focus artifacts is proportional to the size and absorption of the artifact-causing features (Svahn et al 2007). In case of hand imaging this problem becomes even more dramatic, because artifacts are produced by bone tissue, which has a high density. Limited angle artifacts reduce the diagnostic value of reconstructed images. Therefore, a lot of effort is made to investigate how to improve tomosynthesis performance and obtain images with higher quality. Characteristics of the reconstructed images depend on the choice of the reconstruction algorithm (Zhang et al 2006, Wu et al 2004) and on the imaging system parameters as well as the geometry (e.g. Machida et al 2010).
The main focus of this paper is to describe and discuss a novel dual-axis tilt acquisition geometry for the imaging of hands. Some preliminary results using a downsampled dataset for the faster calculation time can be found in Levakhina et al (2012). In this paper the system is analyzed in details, including the validation of the simulation setup and extensive simulation studies of several geometry parameters for both, the traditional scanning geometry and the proposed tilt geometry. The paper is organized as follows. In the second section a literature review on how the performance of tomosynthesis with traditional scanning geometry depends on geometry parameters is presented. This is done to detect the limitations of traditional geometry. Later, this is used to show that our simulation setup is sensitive enough to reproduce those results and therefore is valid to use it for the evaluation of the proposed geometry. The novel acquisition geometry and its theoretical aspects are presented in section 3. The simulation setup is described in section 4. Section 5 presents results and discussion. It consists of two parts. In the first part the standard geometry is simulated and in the second part the proposed dual-axis tilt geometry is simulated. The conclusions section summarizes the paper.
2. Effect of system acquisition parameters: a literature review
A number of investigators have analyzed the tomosynthesis system performance and the impact of acquisition parameters on clinical image quality. This section gives a literature review on the effect of tomosynthesis acquisition parameters in case of classical tomosynthesis geometry. The following parameters are considered: the angular range (sweep angle), the number of projections and the angular step size (projections density).
2.1. Varying the angular range of an x-ray tube rotation
The angular range of an x-ray tube rotation, also known as a sweep angle, denoted by θ, is the size of the total arc above the rotation center and determined by an x-ray tube position of the first and the last measured projection. The angular range in clinical applications is typically between 20° and 50°.
One of the first studies discussing the impact of acquisition parameters on image quality in DT showed that an increase of the angular range leads to a narrower z-spread, i.e. it reduces the slice thickness (Li et al 2004, 2006). Several further studies also showed that extending the angular range leads to sharper in-focus features and increased depth resolution (Deller et al 2007, Kontos et al 2008, Mertelmeier et al 2008). This is in accordance with observed measurements of a small high-contrast steel bead (Hu et al 2008a, 2008b). A simulation study, which includes an empirical system response characteristics also agrees that increasing the angular range always improves the axial resolution (Sechopoulos and Ghetti 2009). Such characteristics as the modulation transfer function (MTF) and the noise power spectrum (NPS) are mainly affected by the angular range (Zhou et al 2007). The lesion detectability (Chawla et al 2009, Reiser and Nishikawa 2010) is also improved when the angular range is increased. The recent works conclude that the imaging parameters should be adapted based on the visualized anatomy. For visualization of large body parts as an abdomen, the angular range can be decreased because the abdomen region does not contain fine structures and the depth resolution in this case is not critical. For the visualization of fine anatomy such as hands or feet, the angular range should be increased in order to gain depth resolution and reduce the risk of missing some subtle structures (Machida et al 2010). It might be challenging to optimize parameters for several different tasks at the same time (Richard and Samei 2010a, 2010b).
2.2. Varying the number of projections
The number of measured projections, acquired over the angular range θ is denoted by Nproj. In clinical applications for breast imaging Nproj typically varies between 10 and 30 (Sechopoulos 2013). For chest imaging Nproj might be up to 60.
A high number of projections results in less reconstruction artifacts, superior image quality and finer texture (Kontos et al 2008, Maidment et al 2006). However, the improvement of axial resolution and the reduction of out-of-focus artifacts tends to saturate when Nproj is beyond a certain threshold which depends on the angular range (Ren et al 2006, Kontos et al 2008, Sechopoulos and Ghetti 2009). Increasing the projection number while keeping the total dose of a scan fixed leads to dose reduction in a single exposure and increases the image noise (Sechopoulos and Ghetti 2009, Chawla et al 2009). Vice versa, increasing the number of projections while keeping the dose per projection the same, i.e. increasing the total dose, decreases image noise (Machida et al 2010).
As a conclusion, the number of projections plays a less important role than the angular range. The improvement based on the larger projection number tends to saturate. The number of projections mainly contributes to the dose and image noise but does not change the appearance of artifacts.
2.3. Varying the angular step size
An angular step size, denoted by Δθ, is defined as the angular range divided by the number of projections minus one. It is described by the x-ray tube position from the current measured projection to the next measured projection. Sometimes an inverse measure, called a projection density, can be found in literature (Deller et al 2007, Machida et al 2010).
It is important to use a reasonably large angular range (e.g. >30°) and to limit the angular step size value (e.g. <2°) (Hu et al 2008a). An insufficient number of projection with large angular separation between views might lead to ringing in the artifact spread function (ASF) (Zhou et al 2007) and results in ripple artifacts (Hu et al 2008a). Decreasing the angular step size for a fixed angular range reduces ripple (Deller et al 2007) and streak (Mertelmeier et al 2008) artifacts and improves the lesions detectability (Reiser and Nishikawa 2010). At the same time, ripple visibility also depends on the thickness of the tissue and the distance to the ripple-causing structures. The projection density should be increased when imaging a thick part of the body such as chest and can be reduced when imaging a thin part of the body (Machida et al 2010).
3. Methods: a hybrid dual-axis tilt acquisition
As discussed in the previous section, the tomosynthesis performance can be improved by adjusting imaging parameters. We propose a novel acquisition geometry, which has been inspired by a solid angle tomosynthesis (Zhang and Yu 2010) and tomosynthesis with specially designed carbon nano-tubes as x-ray sources (Qian et al 2012). Here, the acquisition is done by positioning the x-ray source in two perpendicular directions or by replacing an x-ray tube by an array of x-ray sources. A similar dual-axis acquisition approach is known from the scanning transmission electron microscopy (Iancu et al 2005, Arslan et al 2006). In this case, the object is tilted in two perpendicular directions using a movable stage.
The hybrid approach proposed in this paper is a composition of the methods mentioned above. The acquisition in one direction is still done by moving the tube along the standard arc trajectory. The acquisition in the additional perpendicular direction is done by tilting the object. The existing device configuration (figure 1(a)) is extended by a tiltable platform, which is mounted on the detector and supports the object. The platform can be tilted in the y-direction orthogonal to the x-ray tube rotation axis (figure 1(b)). Instead of taking one image, several digital images are taken at each x-ray tube position, differing in the tilt angle of the platform. In order to avoid motion artifacts, the anatomy must be fixed. This can be done using e.g. velcro-strips and rubber bands. Additionally, soft foam can be used as padding for comfortable hand positioning. In order to avoid unwanted structures in the image, the tilting mechanism must be positioned outside the detector sensitive area.
Figure 1. A hybrid dual-axis tiltable geometry. The x-ray tube moves along an arc in a stop-and-shoot mode. Instead of taking one image, several images are taken at each stop position, while the object is tilted. (a) Tomosynthesis geometry. (b) Tiltable platform.
Download figure:
Standard image High-resolution imageTilting the object is geometrically equivalent to moving the x-ray tube outside the standard arc trajectory. This allows for acquiring additional projections by collecting information about an object from the sides. This way, for each position of the x-ray tube several projections outside the standard arc are acquired without moving the tube in the perpendicular direction. At the same time, the number of projections in the original direction is reduced. The total number of projections and therefore the applied dose stays the same (
).
The proposed geometry allows for capturing more singularities of the Radon transform (Quinto 1993), filling more data in the Fourier space and better approximating the Tuy–Smith conditions (Tuy 1983, Smith 1985). In the following subsection three different aspects of limited data are described more in detail.
3.1. Singularities of Radon transform and limited data
Let a direction vector θ belong to a unit sphere θ ∈ Sn − 1 and be a hyperplane through the origin perpendicular to θ. Let a line l(θ, s) in
be parametrized by a direction θ and a distance s from the origin. The Radon transform R of a function
is defined as a line integral over a hyperplane, which is perpendicular to θ with signed distance s from the origin:

For the properties of the Radon transform see e.g. Faridani (2003) or Quinto (2006).
The tomosynthesis reconstruction problem is to reconstruct the function f based on Rf acquired on a restricted subset of a unit sphere . Uniqueness of the solution requires an infinite number of lines be measured. In practice, only a finite number of lines can be measured. Reconstruction from the real limited angle data is more ill-posed than reconstruction from the real complete angle data (Quinto 1993). A unique solution exists but it is unstable. Only certain features of the object can be reconstructed.
A singularity of the object is defined as a density jump between two materials, i.e. where the density function is not smooth. Singularities of the object are closely related to the singularities of the Radon transform. A singularity of the object produces a singularity in the Radon transform only if a line integral is measured in the direction perpendicular to this singularity. Such singularities are called visible and can be stably reconstructed (Quinto 1993, 2007). Other singularities are called invisible and cannot be stably reconstructed. In the three-dimensional case, the x-ray tube at each position casts a so-called visible ring on a sphere object. The orientation of the visible ring depends on the xy position and height z of the sphere. An arc acquisition trajectory AB results in a set of visible rings, see figure 2(a). All rings crosses in two opposite points. All other points on the sphere, denoted by dashed line remains invisible and cannot be reconstructed stably. If the x-ray tube is moved to the point C, which is located in the perpendicular plane, a visible ring C1C2 with an essentially new orientation will be produced, see figure 2(b). The acquisition along the perpendicular arc will make visible additional regions. To summarize, the dual-axis acquisition geometry allows for capturing singularities which are located in perpendicular planes (i.e. in the plane of new virtual x-ray tube movement) and would have stayed invisible in case of arc trajectory.
Figure 2. (a) An acquisition along the one-dimensional arc results in a set of visible rings of singularities. (b) If the x-ray tube is moved to the point C, which is located in the perpendicular plane, a visible ring C1C2 with an essentially new orientation is produced. (a) Set of visible rings for the acquisition along AB. (b) A new visible ring casted in the position C.
Download figure:
Standard image High-resolution image3.2. Incomplete Fourier space
According to the well-known Fourier slice theorem, limited angle tomosynthesis acquisition along an arc θx covers only a limited wedge θx in the Fourier domain. The limited wedge results in the limited axial resolution. Dual-axis tilt acquisition geometry allows for filling an additional wedge in the xz-plane. A schematic representation of the measurement process and the Fourier space are shown in figure 3. An arc AB in the image domain and the corresponding wedge θx = ∠AOB = (∠AOB)ω in the Fourier space are related to the standard acquisition mode. An additional arc CD and a wedge θy = ∠COD = (∠COD)ω belong to the hybrid dual-axis tilt acquisition. Not only a wedge is filled in Fourier space, but a cylinder with missing cone along z-direction. It shows that more data, which can be used for reconstruction, became available.
Figure 3. Illustration of the incomplete Fourier domain. Only the wedge θx = ∠AOB = (∠AOB)ω is covered by the standard acquisition mode. With the dual-axis acquisition both wedges, θx = AOB = (∠AOB)ω and θy = ∠COD = (∠COD)ω are obtained. (a) Image domain. (b) Fourier domain, dual-axis mode.
Download figure:
Standard image High-resolution image3.3. Tuy–Smith sufficiency condition
The Tuy–Smith sufficiency condition (Tuy 1983, Smith 1985) states that if on every plane that intersects the object there exists at least one cone-beam source point, then one can reconstruct the object exactly. The standard tomosynthesis trajectory does not fulfil this condition. With additional data acquired outside the standard arc trajectory, more planes through the object intersect the x-ray tube trajectory. Therefore, the degree of violation of the Tuy–Smith sufficiency condition is decreased, but the condition is still not satisfied.
4. Materials: tomosynthesis simulator and reconstruction software
Most studies on tomosynthesis performance, which can be found in literature use characteristics, which can be easily described mathematically, calculated and measured using technical phantoms rather than anthropomorphic phantoms. For example, resolution and artifacts are typically measured using special resolution phantoms with sharp edges and described by the MTF, point spread function, NPS, ASF or signal-to-noise ratio (SNR). But it is not straightforward to apply those metrics when imaging real objects. Clear edges for MTF calculation or large homogeneous features for SNR calculation are not always present in real objects. Typically, anthropomorphic phantom reconstructions are evaluated only visually or based on the observer studies. The simulation software allows for measuring image quality based on one quantitative parameter (reference-based metric), using the whole reconstruction volume at once (e.g. not just a single slice, a region of interest (ROI) or one specific feature) and using more realistic objects than the typically used technical phantoms.
For the current study, a software simulation framework for tomosynthesis acquisition and reconstruction has been developed using the geometry of the Siemens Mammomat Inspiration device. The device is equipped with a large flat-panel detector and a half-cone x-ray tube. The angular range, which is used in clinical routine in this device is 50° and the number of the acquired projections is 25. A typical hand size is about 220 × 130 mm with a thickness in a range of 30–50 mm. The size of the bone phantom (described in the next section) is approximately 20 × 10 mm and the thickness is 6 mm. The geometry of the device has been scaled down by a factor of 5 due to the physical dimensions of the used phantom. This grants a proportionality between the simulations and the true dimensions of a real hand object. Images are reconstructed parallel to the detector plane with a slice thickness of 0.2 mm and a pixel size of 0.017 mm. The imaging parameters are summarized in table 1.
Table 1. Tomosynthesis geometry and imaging parameters.
System parameter | Value | |
---|---|---|
Distances | Source-to-Iso | 121.7 mm |
Iso-to-table | 8.2 mm | |
Table-to-detector | 4.8 mm | |
Detector | Detector length x | 60.9 mm |
Detector length y | 47.8 mm | |
Dexel size | 0.017 mm | |
Reco | Slice size | 3584 × 2816 |
Pixel size | 0.017 mm | |
Slice thickness | 0.2 mm |
An ideal system was considered and no physical limitations were taken into account. The x-ray source is a point source and the x-ray tube spectrum is assumed to be monoenergetic. The projections have been simulated without noise. The forward and backprojections have been modeled using the distance-driven method (De Man and Basu 2004) adapted for the fixed-detector tomosynthesis geometry (Levakhina et al 2011).
The simulator allows for simulating two geometries: a standard acquisition geometry and a hybrid dual-axis acquisition geometry. The geometry with fixed detector, lying in the xy-plane and the x-ray tube, which moves along a one-dimensional arc around the x-axis above the detector is referred to as the standard acquisition mode. The geometry when additional data is acquired outside the one-dimensional arc is referred to as the dual-axis acquisition mode.
4.1. A digital finger bone phantom
A digital high-resolution three-dimensional volume of a dried human finger bone4 has been used as phantom for the projections simulation and as a reference for the quantitative evaluation of the results. The dataset has been acquired with a Skyscan1172 micro-CT and reconstructed with the FDK reconstruction algorithm (Feldkamp et al 1984). The reconstruction has been post-processed using denoising and background segmentation. Slices in z-direction have been averaged for the slice thickness of 0.2 mm resulting in 30 slices.
Three orthogonal slices of the bone volume are shown in the figure 4. The bone dataset represents the anatomy of fine trabecular structures, which are of the interest for tomosynthesis hand imaging. The selected ROIs are marked with ellipses. The ROI1 represents an in-plane region without tissue to show out-of-focus artifacts. ROI2, ROI3 and ROI4 represent a bone boundary in the in-plane slice and two orthogonal slices, respectively.
Figure 4. A digital bone phantom based on the micro-CT FDK reconstruction. Three orthogonal slices are shown. Four regions of interests are marked with ellipses. An orientation angle with respect to the x-ray tube rotation axis is 45°.
Download figure:
Standard image High-resolution imageFor the object orientation study, the phantom can be rotated in the xy-plane. The object orientation angle is considered as the angle between the main axis of the object and the y-axis. In figure 4 the orientation angle is 45°.
4.2. Simultaneous algebraic reconstruction technique (SART)
The simulation of the hybrid dual-axis tilt acquisition geometry results in data acquired along a non-standard trajectory. These projection data have to be reconstructed. It can be done using iterative reconstruction algorithms, which are known to be flexible with the respect to the imaging geometry. Independent of the acquisition geometry and the trajectories, the tomographic reconstruction problem can be represented as a system of linear equations . Here,
is a discrete representation of the unknown distribution of x-ray attenuation coefficients within the scanned volume,
is a vector of the measured projection data and
is a system matrix, which accounts for the system geometry. The system matrix is very large and sparse. The number of non-zero elements for the three-dimensional tomosynthesis problem can be up to several hundred Gb. Therefore, the system matrix must be calculated on-the-fly. The SART searches for a solution by minimizing a weighted least-square function and results in the following update scheme:
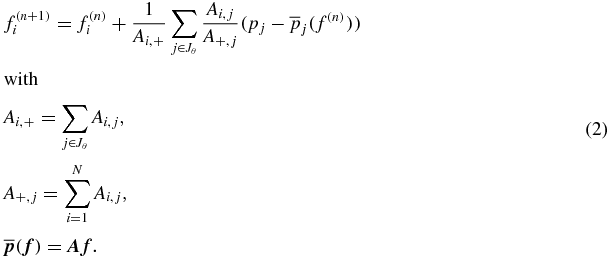
In this equation, Ai, + is a normalization factor based on the number of rays intersecting each voxel, A+, j is a normalization for the path through all voxels in the current ray and is the forward projection of the current guess. The updating term is applied after one projection view j ∈ Jθ has been processed.
4.3. Image quality metrics for performance evaluation
In this study, the performance of tomosynthesis in dependency of the imaging parameters and system geometry has been evaluated quantitatively and qualitatively.
4.3.1. Quantitative reference-based evaluation
The reconstructed volumes have been quantitatively evaluated using the well-known normalized root mean squared error (NRMSE). The NRMSE has been used to measure the similarity degree between the digital reference phantom and the reconstructed tomosynthesis volume. The NRMSE is given by equation (3). Here, the is the digital phantom volume used as a reference, f = (f1, ..., fN)T ∈ RN is the reconstructed volume corresponding to the simulated projections using the selected acquisition parameters.

The smaller the NRMSE, the smaller the difference between the reconstructed volume and the reference phantom.
4.3.2. Qualitative visual-based evaluation
Additionally, in the current study a qualitative visual-based inspection and comparison of the reconstructed slices has been carried out. Qualitative visual inspection is an important assessment because it is difficult to capture artifact specificity and quantify image quality using only one number (e.g. Bian et al 2010). The overall visual inspection and comparison of in-plane and two orthogonal axial slices has been performed with focus on the four selected ROIs, shown in the figure 4.
5. Results and discussion
In this section the system geometry parameters are analyzed. The results for the geometry with traditional scanning trajectory reproduce the state-of-the-art results given in the literature review and give an insight into the limitations of the standard geometry. It will be shown that the proposed dual-axis acquisition geometry is superior and can overcome these limitations.
5.1. Standard acquisition mode
In standard acquisition mode the x-ray tube moves along a one-dimensional arc trajectory. Acquisitions have been simulated according to the chosen parameters. The obtained projection data have been reconstructed using SART. Then the reconstruction results have been compared to the original phantom. The reconstructed volume is visualized with three pre-selected orthogonal slices. The reference is shown in the figure 4. The selected ROIs are marked with ellipses.
5.1.1. Influence of the number of projections Nproj
The influence of the number of projections has been studied for three fixed angular ranges. The number of projections has been varied from 5 to 100. It is important to note that the dose is kept constant per projection, since no noise is simulated in this study. Therefore, results cannot be directly transferred into clinical data acquisition conditions. In figure 5(e) the NRMSE between the reconstructed volume and the reference data is plotted against Nproj. Here, triangle markers denote data corresponding to an angular range of θ = ±25°, which is used in clinics (according to the Siemens Mammomat Inspiration geometry). The diamond and circle markers indicate acquisitions recorded with the halved (θ = ±12°) and the doubled (θ = ±50°) angular range, respectively. The data show an improvement in image quality when increasing the number of projections. However, the gain of image quality saturates for large values of Nproj. After a certain value there is a plateau and further increase in projection number does not result in further visible improvement. The saturation values are marked with short vertical lines. For example, the saturation value for an angular range of ±25° is 25.
Figure 5. Simulation results with varying number of projections Nproj. The reconstruction of the finger bone (a)–(d) for the fixed angular range θ = ±25° are highlighted with bold markers in the quantitative evaluation plot (e). The saturation values in plot (e) are marked with vertical lines. (a) Nproj = 3, (b) Nproj = 12, (c) Nproj = 25, (d) Nproj = 50 and (e) NRMSE between the simulation and the reference.
Download figure:
Standard image High-resolution imageSelected reconstruction results for θ = ±25° and Nproj = 3, 12, 25 and 50 are shown in figures 5(a)–(d). The data points corresponding to the shown reconstruction results are highlighted with bold markers in figure 5(e). The reconstructed images visually illustrate the curves behavior. The image obtained with Nproj = 3, shown in figure 5(a), is blurry and contains only few details. When increasing Nproj to 12 (figure 5(b)) and then to 25 (figure 5(c)), more details become visible. However, no further improvement can be noticed when increasing Nproj from 25 to 50 (figure 5(d)). The values Nproj = 25 to Nproj = 50 correspond to the plateau region in the plot (figure 5(e)). In summary, when increasing the number of projections, the out-of-focus artifacts in the ROI1 are slightly reduced. The bone boundary in the in-plane ROI2 is better recovered, however the bone boundaries in the axial slices in ROI3 and ROI4 are still blurred and hardly recognizable. Additionally, in a clinical case, the increase in the number of measured projections means an increase in patient dose if the dose per projection is kept constant. If the total patient dose is constant it will result in more noise in each projection and a potentially noisier reconstruction.
As a conclusion, an increase of the projection number is sensible only up to a certain value, which depends on the angular range. This saturation value is between 15 and 30 projections for a wide range of angular ranges between θ = ±12° and θ = ±50°.
5.1.2. Influence of the angular range θ
The influence of the angular range has been studied for five fixed numbers of projections. The angular range has been varied from θ = ±5° to θ = ±75°. Angles larger than ±75° become infeasible because of the fixed detector geometry (values will be projected outside the detector sensitive area).
In figure 6(e) the NRMSE between the obtained reconstruction and the reference is plotted against the angular range θ. The curve with triangle markers describes Nproj = 25, which is used in clinical examination. The overall behavior of the curves indicates improvement in image quality when increasing the angular range. The gain in quality is greater for a large number of projections (Nproj = 50–100), compared to a small number of projections (Nproj = 6–12). For small projection numbers image quality decreases again after reaching a minimum indicated by vertical lines in figure 6(e). The minimum is found at ±55° and ±60° for Nproj = 6 and Nproj = 12, respectively. Image quality decreases again because the spacing between projections becomes too large, resulting in ripple artifacts (blurring does not occur anymore).
Figure 6. Simulation results for varying angular range θ. The reconstructions of the finger bone (a)–(d) for a fixed number of projections Nproj = 25 are highlighted with bold markers in the quantitative evaluation plot (e). The values of angular range when image quality decrease due to ripple artifacts are marked with vertical lines. (a) θ = ±12°, (b) θ = ±25°, (c) θ = ±50°, (d) θ = ±75° and (e) NRMSE between the simulation and the reference.
Download figure:
Standard image High-resolution imageThe reconstruction results for Nproj = 25 and θ = ±12°, ±25°, ±50°, ±75° are shown in figures 6(a)–(d). The images illustrate the quality improvement when increasing the angular range. The image obtained with θ = ±12° (figure 6(a)) shows blurring artifacts in ROI1 and lost bone boundaries in ROI2-ROI4. The image obtained with θ = ±75° (figure 6(a)) shows much less blur in ROI1 and bone boundaries recovery in both, the in-plane ROI2 and axial ROI3 and ROI4. The general appearance of the image in figure 6(a) is very similar to the reference image (figure 4). An increase of θ does not result in higher dose if the projection number is fixed. However, if the number of projections is insufficient, image quality decreases.
As a conclusion, the angular range θ plays an important role for the quality of the reconstructed images, which is increasing with increasing θ. At the same time, if the spacing between projections becomes too large, image quality decreases.
5.1.3. Influence of the angular spacing Δθ
The influence of the angular step size has been studied for different combinations of the angular range θ from ±5° to ±50° and for varying the number of projections Nproj (5 to 50). This results in various angular step sizes from Δθ = 0.5° to Δθ = 15°. Results are shown in figures 7(d) and (e). The dependence of the angular step size for different pairs of θ and Nproj is shown in figure 7(d). The NRMSE between the simulated and the reference data for the chosen pairs (θ, Nproj) is shown in figure 7(e). Results are represented as contour lines. When following a contour line with fixed Δθ in figure 7(d), the image quality increases (see figure 7(e)) for increasing the angular range and the number of projections.
Figure 7. Simulation results for varying the angular step size Δθ. The reconstructions of the finger bone (a)–(c) for the fixed angular step size Δθ = 2° and different θ and Nproj are highlighted with bold markers in the quantitative evaluation plots (d) and (e). (a) Nproj = 12, θ = ±12°, (b) Nproj = 25, θ = ±25°, (c) Nproj = 50, θ = ±50°, (d) Δθ contour plot and (e) NRMSE contour plot.
Download figure:
Standard image High-resolution imageThe overall behavior of the curves summarizes the conclusions made in two previous subsections. Increasing the number of projections with fixed angular range (i.e. increasing projection density) helps to improve image quality at larger angular ranges and saturates for smaller angles. The angular range plays a more important role than the projection number. Increasing the angular range with a fixed number of projections improves the image quality.
5.2. The dual-axis tilt acquisition mode
Two simulation studies have been carried out to evaluate the dual-axis acquisition. In the first study the influence of the angular range in x-direction and a pattern of the projection re-distribution has been studied. In the second study the influence of number of projections for selected 'symmetrical' distribution scheme has been carried out. The comparison between the dual-axis acquisition geometry and the standard acquisition geometry has been done using a fixed number of projections, i.e. the dose is constant. Additionally, the influence of the object orientation has been studied for both geometries.
5.2.1. Influence of the angle in x-direction and re-distribution of projections
The influence of the angle in x-direction has been studied using an angular range in the original direction θy = ±25° and the fixed number of projections. The angle in x-direction has been varies from θx = ±2° to θx = ±25°. A fixed number of projections can be re-distributed in two perpendicular directions in several fashions. If the number of projections Nproj = 24, they can be re-distributed as following: Nproj, y = 6 and Nproj, x = 4, Nproj, y = 8 and Nproj, x = 3, Nproj, y = 12 and Nproj, x = 2. Additionally, if the root of the total number of projection is a natural number, they can be redistributed 'symmetrically' Nproj, x = 5, Nproj, y = 5. We are aware of the fact that for the 'symmetrical' pattern Nproj is 25. However, we would like to test all possible patterns and based on the experiments with standard geometry presented in the previous section, we assume that the results obtained using Nproj = 24 and Nproj = 25 are fairly comparable with each other. For the comparison, the standard acquisition geometry has been simulated using θ = ±25°, and Nproj = 25 based on the geometry of the Siemens Mammomat Inspiration. The simulation results are presented in figure 8(e). The horizontal line with ○-markers corresponds to the standard mode with no additional angle in x-direction. When the value of θx is small (±2°) and the Nproj, x = 2, the dual-axis geometry performs slightly better than the standard geometry. When the number of projections in x-direction is increased, the image quality of the dual-axis mode becomes even worse than the one standard geometry. It happens because an increase of Nproj, x means decrease of Nproj, y, which, in turn leads to the decrease in image quality. This effect can be clearly seen in the reconstructed images in figures 8(a) and (b). In both images the bone boundaries in ROI2–ROI4 are lost and out-of-focus blurring is strongly present. All curves in the plot show the main tendency of improving the image quality when larger θx is used for all projection re-distribution schemes. When θx is relatively large (±25°) and it is value is equal θy, the 'symmetrical' re-distribution scheme performs the best, compare figures 8(c) and (d). In both images the bone boundaries in ROI2–ROI4 are recovered, however in figure 8(c) one can see the noticeable residual blurring in ROI1. At the same time, multiple tilting steps of the object over the wide angular range might be practically difficult.
Figure 8. Simulation results for varying angular range in x-direction and different re-distribution of projections for dual-axis geometry. The reconstructed slices of the finger bone (a)–(d) are highlighted with bold markers in the quantitative evaluation plot (e). The ○-markers correspond to the standard mode with no angle in x-direction. (a) Standard mode, θx = ±0°, Nproj = 25. (b) Dual-axis, θx = ±2°, Nproj, x = 5, Nproj, y = 5. (c) Dual-axis, θx = ±25°, Nproj, x = 2, Nproj, y = 12. (d) Dual-axis, θx = ±25°, Nproj, x = 5, Nproj, y = 5. (e) NRMSE plot.
Download figure:
Standard image High-resolution image5.2.2. Influence of the projection number
To evaluate the influence of number of projections, the best performance parameters from the previous experiment (previous section) have been taken. The dual-axis tilt mode has been simulated using θx = θy = ±25° and the symmetrical projection re-distribution. Four different numbers of projections have been tested. The following values have been selected Nproj = 16, 25, 36, 49, because their roots are natural numbers. In the symmetrical projection re-distribution scheme the number of projection per each axis in the tilt geometry is a root of the total number of projections in the standard geometry. Therefore, the corresponding dual-axis tilt mode has been simulated using θx = θy = ±25° and = Nproj, y = 4, 5, 6, 7 which is equivalent to a total projection number Nproj of 16, 25, 36, 49 in standard mode.
The simulation results are presented in figure 9(e). The line with diamond markers corresponds to data using standard acquisition mode and the line with triangle markers correspond to data gained in dual-axis tilt acquisition mode. The NRMSE is much smaller when using the dual-axis tilt acquisition mode in all tested cases compared to the standard acquisition mode. The image quality is improved when more projections are used for both acquisition modes.
Figure 9. Simulation results for the acquisition with and without tiltable platform (a)–(d) and the quantitative evaluation plot (e). The ▵-markers correspond to the standard mode and the ◊-markers correspond to the dual-axis mode. The standard mode parameters are: θx = ±0°, θy = ±0°. The dual-axis mode parameters are: θx = ±25°, θy = ±25°. (a) Standard mode, Nproj = 16. (b) Dual-axis, Nproj, x = Nproj, y = 4. (c) Standard mode, Nproj = 25. (d) Dual-axis, Nproj, x = Nproj, y = 5. (e) NRMSE plot.
Download figure:
Standard image High-resolution imageThe reconstruction results with Nproj = 16 and 25 in standard mode are presented in figures 9(a) and (c). This is equivalent to Nproj, y = Nproj, y = 4 and 5 in the dual-axis mode. The corresponding images are presented in figures 9(b) and (d). The images illustrate notable image improvement when using dual-axis mode. The images obtained with standard acquisition mode (figures 9(b) and (c)) are affected by blurring due to out-of-focus artifacts in ROI1. Bone boundaries in in-plane ROI2 and axial ROI3 and ROI4 are lost. The corresponding images obtained with the tiltable platform (figures 9(b) and (d)) show less out-of-focus artifacts. The bone boundaries are recovered in ROI2–ROI4.
As a conclusion, using the same number of projections (i.e. the fixed total dose), the dual-axis geometry provides images with better quality compared to the standard acquisition mode. The out-of-focus artifacts are reduced and the in-plane and axial boundaries of the bone are restored.
5.2.3. Influence of the object orientation
The influence of the object orientation with respect to the x-ray tube rotation axis has been studied for the standard mode and the dual-axis acquisition mode. The standard acquisition mode parameters are: θ = ±25°, Nproj = 25. The dual-axis acquisition mode parameters are: θx = θy = ±25°, Nproj, x = Nproj, y = 5. The NRMSE, measured for all possible object orientations from 0° to 360° is presented in figure 10. The curve corresponding to the standard mode is denoted with diamond markers and the curve corresponding to the dual-axis mode is denoted by triangle markers. In standard acquisition mode image quality decreases when the orientation angle is close to 90° or 270°. The loss in image quality manifests itself as peaks in figure 10, indicated by arrows. The dual-axis acquisition mode does not show those peaks, and, therefore, reduce the dependency of image quality on the object orientation. In addition, the image quality for the dual-axis acquisition mode is better than in the standard mode for all object orientations.
Figure 10. Influence of object orientation on image quality. A standard acquisition mode is indicated with the ▵-markers and the dual-axis acquisition mode is indicated with the ◊-markers. Image quality decrease in the standard mode is marked with black arrows.
Download figure:
Standard image High-resolution image6. Conclusion
In this work the performance of the novel acquisition geometry for musculoskeletal tomosynthesis and influence of several imaging parameters have been studied. The experiments have been carried out using a software simulation and a software bone phantom created based on the micro-CT scan (FDK reconstruction). Simulated projections have been reconstructed using SART, which provides flexibility with respect to the acquisition geometry. The experiments with traditional acquisition geometry have been done to validate the used simulation framework. The results show the same tendency as it can be found in literature. It shows that the simulation setup is sensitive enough to capture differences in image quality for different geometry parameters.
Analysis of the imaging parameters has shown that an increase in the number of projections improves the image quality until a certain saturation value, which depends on the angular range. The saturation value lies in such region that image quality is improved, but the out-of-focus blur is still present and bone boundaries are still not sharp enough to recognize them. The angular range has more influence on the image quality. The increase of the angular range improves image quality. If the angular step size between projections becomes too large, image quality decrease again. Therefore the angular step size (or projection density) also plays an important role. With the fixed angular step size, images are better, when a larger angular range is used. Is important to note this study has several limitations. First, noise is not considered. Second, in a clinically relevant study, one would compare the effect of imaging parameters such as Nproj by keeping the patient dose constant. Third, in this study the imaging of hands is considered, but the dried bone specimen does not fully represent the hand anatomy with soft tissue. Finally, although it was shown that the NRMSE measure can be used to compare images, it is a general measure and cannot give detailed information about image quality parameters such as sharpness, contrast and SNR.
The novel hybrid dual-axis tilt acquisition principle acquires the data outside the standard one-dimensional arc trajectory, which is typically used in clinical practice. The proposed geometry allows for capturing more singularities of the Radon transform, filling more data in the Fourier Space and better approximating the Tuy–Smith condition. New data is aquired using a standard device configuration with additional tiltable object-support platform, mounted on the detector. Acquisition in one direction is still done by moving the tube along the standard arc trajectory and acquisition in the additional perpendicular direction is done using the tiltable platform. The object is tilted in direction, perpendicular the x-ray tube movement, in several predefined positions for each position of the x-ray tube. This way, for each position of the x-ray tube several projections outside the standard arc are acquired without additional movement of the tube in the perpendicular axis.
The hybrid dual-axis tilt acquisition principle provides better in-plane and axial image quality using the same number of projections (i.e. the same dose) and without major modification of the existing tomosynthesis devices. For the small values of the additional angle in x-direction, relatively small number of projections should be re-distributed to the x-axis. When the value of the angle in x-direction becomes comparable to the value of the angle in the original y-direction, projections should be re-distributed symmetrically to achieve the best results. One possible shortcoming is the fact that maximum simulated angle of ±25° might be difficult to use in other applications than imaging of hands (e.g. in breast imaging). The dual-axis acquisition geometry always outperforms the traditional scanning geometry when a fixed number of projections is used. Additionally, it has been shown that in the standard acquisition mode, the image quality depends on the object orientation with respect to the x-ray tube rotation axis. The tiltable platform mode reduces the influence of the image orientation with respect to the x-ray rotation axis on the image quality. Tilting the object is geometrically equivalent to moving the x-ray tube outside the standard arc trajectory. The standard device can be easily extended because no major modifications or additional space for orthogonal tube movement is needed.
Acknowledgments
This work was supported by the Graduate School for Computing in Medicine and Life Sciences funded by Germany's Excellence Initiative (DFG GSC 235/1).
Footnotes
- 4
The human cadavers—respectively bodies/heads/arms/legs feet etc as parts of cadavers—were used and dissected in this examination under permission of the 'Gesetz ueber das Leichen-, Bestattungs- und Friedhofswesen (Bestattungsgesetz) des Landes Schleswig–Holstein vom 04.02.2005, Abschnitt II, 9 (Leichenoeffnung, anatomisch)'. In this case it is allowed to dissect the bodies of the donators (Koerperspender/in) for scientific and/or educational purposes.