Abstract
X-ray diffraction, specific heat, magnetic susceptibility and inelastic x-ray scattering measurements on the transurarium oxypnictides NpFeAsO and NpFeAsO0.85F0.15 are presented. No superconductivity down to 2 K was observed upon fluorine doping, contrary to the structurally analogous rare-earth pnictides. No modification of the phonon density of states was observed upon doping with fluorine. We discuss our results in light of the latest experimental and theoretical studies on the role of phonons in the superconducting pnictide compounds.
Export citation and abstract BibTeX RIS
1. Introduction
Despite the explosion of research in the past few years, our understanding of the magnetism and superconductivity in the high-temperature iron-based superconductors is still far from complete [1]. Are the magnetic interactions strongly correlated and local, or weakly correlated and itinerant? What is the relationship between the magnetic and superconducting phenomena? What is the extent to which one can make analogies between the high-temperature superconducting cuprates, in which magnetism and superconductivity also have an apparently symbiotic relationship?
The iron-based superconductors all share quasi-two-dimensional layers of iron which are tetrahedrally coordinated with As, Se or Te. In the RFeAsO '1111' family, single layers of FeAs are separated by R–O layers, where R = rare earth element. All of the rare-earth 1111 parent compounds present both structural and magnetic phase transitions around 150 K. Upon electron doping (performed by making the compounds oxygen deficient or substituting fluorine for oxygen) both of these transitions are suppressed and a superconducting regime is found, with the maximum superconducting transition temperature of 55 K found in doped SmFeAsO [2] and NdFeAsO [3]. The 1111 family contains the maximum superconducting transition temperature found in any bulk iron-based superconductor to date.
The role that nematic order may play in the superconductivity in the iron-based superconductors is of great interest. It has been suggested that nematic ordering in the electronic degrees of freedom may be the driving force behind the tetragonal-to-orthorhombic transition found in the parent compounds [4]. The importance of nematicity in these systems has been highlighted in a recent investigation of detwinned BaFe2As2 samples using inelastic neutron scattering [5], in which it was demonstrated that the magnetic excitations can break the tetragonal symmetry even when the system has a tetragonal crystal structure.
Published high-resolution inelastic x-ray scattering (IXS) measurements have demonstrated that there is a significant change in the phonon density of states (phDOS) as a function of fluorine doping in NdFeAsO1−xFx, with a clear renormalisation of the phonon energies around 23 meV [6]. Similar behaviour has also been reported in SmFeAsO1−xFx [7]. However complementary x-ray scattering studies on LaFeAsO1−xFx [8] and PrFeAsO1−xFx [9]9 have shown much smaller changes (<1 meV) in the phonons due to doping. A Density Functional Theory (DFT) study [10], which employed a supercell approach to model the effect of fluorine doping in the RFeAsO system, proposed that the fluorine doping primarily acts to change the lattice parameters, resulting in the phonon energy changes observed using IXS. Surprisingly they found that the effective electronic doping of the Fe–As layers upon fluorine doping was rather small. A more recent DFT study [11] also supports the understanding that the fluorine doping primarily causes structural distortions which modify the electronic structure, thereby suppressing the spin density wave and helping to stabilise the superconducting state.
The influence of the spacer layer on iron-based superconductivity—beyond it providing a means of chemical doping the system—has been overlooked by many to date. Part of the reason for this is because in most cases the spacer layer is insulating and therefore does not directly provide additional electronic states at the Fermi level. It has been proposed that if the spacer layer is metallic, interlayer coupling between the electronic states in the spacer layer and in the FeAs layer can actually enhance [12]. However up to now it has only been possible to study this behaviour in systems in which (i) additional 5d electronic states from the spacer layer have been present at the Fermi level [13, 14] (from Pt or Ir) and (ii) the crystallographic structure is very complicated.
Here we provide an alternative way to probe the influence of the spacer layer on iron-based superconductivity: by replacing the rare-earth element in RFeAsO with neptunium, a transuranic (5 f) element. The transuranic elements are ideal candidates for such an investigation, due to the similarities in the ionic radii with the lanthanides which they are replacing, and the fact they are commonly trivalent in similar compounds. This method represents a different means of influencing the correlations in this family of compounds, and potentially extends the rich physics of the iron pnictides. However the radioactive and toxic nature of the actinides makes the already non-trivial fabrication of pnictide compounds even more difficult.
Despite these difficulties, both the neptunium and plutonium members of the 1111 family have been successfully synthesised [15, 16]. It has been shown [15] that in the parent compound of the neptunium family, NpFeAsO, the Np atoms order antiferromagnetically at K, with the wavevector of the magnetic ordering along the c* axis. This ordering wavevector does not break the tetragonal symmetry (unlike the in-plane stripe-like magnetic order found on the Fe atoms in the rare-earth pnictides), and consequently there is no associated structural tetragonal-to-orthorhombic distortion. An upper limit of 0.3
on the ordered Fe magnetic moment in NpFeAsO was also found [15]. It should be noted that this upper limit does not exclude the possibility of Fe magnetic order, especially considering the low ordered Fe magnetic moment of 0.25(7)
which has been found in LaFeAsO samples [17].
Here we present a study of fluorine-doped NpFeAsO, and relate our results to the published results on the parent compound NpFeAsO [15]. We present our IXS measurements on the phDOS in undoped and fluorine-doped NpFeAsO, together with x-ray diffraction, magnetic susceptibility and specific heat measurements on fluorine-doped NpFeAsO. We observed no superconductivity down to 2 K in fluorine-doped NpFeAsO, and the antiferromagnetic order at low temperatures persists in the doped compound. The Néel temperature is suppressed slightly upon fluorine doping and, as observed in the majority of the rare earth analogues, the c lattice parameter is reduced with doping. The phonon density of states in NpFeAsO is found to be independent of fluorine doping. Our work suggests that the existence of nematic magnetic fluctuations may be a requirement for enabling superconductivity in the iron pnictide superconductors [4], as it has been previously found that the magnetic order in NpFeAsO does not impact upon the tetragonal symmetry [15] and in this work we show that no superconducting transition has been observed in NpFeAsO0.85F0.15.
2. Experimental details
Polycrystalline samples with nominal composition NpFeAsO1−xFx (x = 0, 0.15) were synthesized by solid state reaction using Fe2O3 (Alfa Aesar 99.997%), FeF2 (Alfa Aesar 99%), elemental Fe (Alfa Aesar 99.998%), and crystals of NpAs as the starting materials. The carefully mixed powder was pressed into a pellet, sealed in an evacuated silica ampoule and heated at 900 C for 48 h, before being furnace quenched. All operations were carried out in a radio-protected glovebox with low oxygen and water concentrations.
The x-ray diffraction measurements were performed by using both a lab-based x-ray system and also by using synchrotron radiation. The lab-based system was a Bruker D8 Advance diffractometer used in the Bragg–Brentano geometry at the Institute for Transuranium Elements in Karlsruhe, Germany. The data shown in the insets of figure 1 were measured on the powder diffraction beamline, ID31, at the European Synchrotron Radiation Facility (ESRF) in Grenoble, France. Further technical details about the ESRF measurements can be found elsewhere [18].
Figure 1. X-ray powder diffractograms of NpFeAsO and fluorine-doped NpFeAsO measured at 300 K with an x-ray wavelength of λ = 0.35 Å. Black ticks denote the position of Bragg reflections due to NpFeAsO or NpFeAsO0.85F0.15, while red ticks denote the position of Bragg reflections resulting from a NpO2 impurity. The insets show the tetragonal (1 1 1) Bragg peak at 10 K and at 300 K (Q = 4 sin(θ)/λ), demonstrating that no tetragonal-to-orthorhombic distortion was observed in either compound.
Download figure:
Standard image High-resolution imageThe constant-field magnetic susceptibility () measurements were performed using a Quantum Design MPMS-7 SQUID magnetometer. Here we define
as
, where
T and
T. A Quantum Design physical property measurement system (PPMS-9) was used to perform the specific heat measurements via the relaxation method. For the specific heat measurements, the samples were completely encased in a heat-conductive resin (STYCAST 2850 FT). The specific heat data were corrected for the additional resin signal by using an empirical relation that was determined in a previous study [19].
The inelastic x-ray scattering (IXS) experiment was performed at ID28 at the European Synchrotron Radiation Facility using the silicon (12 12 12) configuration at 23.725 keV, which provides an instrumental energy resolution of 1.3 meV, independent of energy and momentum transfer. The measurements were performed in transmission geometry at ambient temperature and pressure. The IXS measurements were performed at 18 different horizontal scattering angles (2) between 28
and 41
, corresponding to a momentum transfer range of 5.8 Å−1 to 8.5 Å−1.
For safety reasons it was necessary to limit the quantity of neptunium on the beamline, but thanks to the small beam size (250 m
60
m) and an attenuation length of approximately 15
m at the x-ray energy used, the amount of sample required was very small. The sample holder was specially designed for this experiment, and is shown in an exploded view drawing in figure 3(b). A photo of the two diamond plate assemblies prepared for the beamtime is shown in figure 3(c). The powdered sample was mixed with toluene and then injected inside a palladium ring. Radiation protection requirements meant that three levels of confinement were required, hence the combination of the diamond plates, the Kapton foil and the aluminium disks. The IXS measurement was possible thanks to the use of single-crystal diamond plates, which were used to encapsulate the sample and were aligned such that they gave a vanishingly small background in the spectral range of interest. The two diamond plates were rotated about the x-ray beam axis to ensure that no Bragg peaks from the diamond windows were near to the horizontal scattering plane. If the measurements had been performed near to a Bragg peak from the diamond, low-energy acoustic phonons from diamond would have contaminated the signal and resulted in a structured background related in a non-trivial way to the diamond phDOS. Our measurement strategy ensured that the measurement would only be sensitive to optic phonons from diamond, which are above 60 meV in energy transfer over more than half the Brillouin zone [20] and are therefore above the range of energy transfers which were studied here.
The inelastic x-ray scattering cross-section for single-phonon scattering can be shown to be proportional to , where Q is the photon scattering vector and
the photon energy transfer [21]):
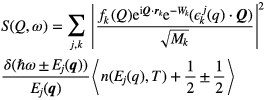
The plus (minus) sign indicates phonon creation (annihilation), and Q = G + q, where G is a reciprocal lattice vector and q is the phonon momentum. The atomic form factor of the kth atom at position rk in the unit cell is defined as f k, Wk is the Debye–Waller factor. The phonon eigenvectors are defined as , and the phonon energies are
. The final term in the expression is the Bose factor.
For IXS studies from powder samples, has to be summed in all directions for each value of momentum transfer Q. It has been shown previously [22] that the summation of many IXS datasets measured at many different values of Q provides a means by which the phonon density of states can be derived. It was also demonstrated that multi-phonon effects (which are not included in the above formalism) can contribute up to 10% of the total IXS signal. However our conclusions in this study are primarily based on a comparison of the IXS data on fluorine-doped NpFeAsO and undoped NpFeAsO, so we deem it unnecessary to remove the multi-phonon signal using the recursive techniques applied in some of the previous studies [6, 7].
3. Results and discussion
Room temperature powder x-ray diffraction data on both NpFeAsO and NpFeAsO0.85F0.15 are presented in figure 1. It should be noted that data shown in this section on the parent compound NpFeAsO corroborates the synchrotron diffraction data presented in an earlier publication [15]. Our x-ray diffraction results show that both compounds have the same tetragonal ZrCuSiAs-type P4/nmm structure as the rare-earth analogues at room temperature. Rietveld refinement of these datasets reveals the structural parameters shown in table 1.
Table 1. The room temperature lattice parameters, fractional positions of the Np and As atoms along the c-axis, and bond angles for NpFeAsO and NpFeAsO0.85F0.15 are shown.
NpFeAsO | NpFeAsO0.85F0.15 | |
---|---|---|
a (Å) | 3.863 69 (4) | 3.863 75 (3) |
c (Å) | 8.361 25 (10) | 8.358 00 (6) |
V (Å3) | 124.82 (1) | 124.77 (1) |
z (Np) | 0.154 77 (14) | 0.154 54 (9) |
z (As) | 0.6709 (4) | 0.6708 (2) |
![]() |
107.0 | 107.1 |
![]() |
5.38 | 4.74 |
![]() |
4.68 | 2.63 |
![]() |
1.32 | 3.26 |
Note: Both compounds present the ZrCuSiAs-type structure, space group P4/nmm (origin choice 2) with Np, Fe, As, and O/F on the 2c, 2b, 2c, and 2a Wyckoff sites respectively.
The insets in figure 1 show that no change in the space group was observed upon cooling in either the parent compound or the fluorine-doped compound down to 5 K. This is different to all the RFeAsO compounds, in which an orthorhombic transition has been observed in the parent compound. While the structural data of the parent and the doped compound are very similar, our data show that the c (out-of-plane) lattice parameter clearly shrinks with fluorine doping. This reduction in the c lattice parameter was also observed in several rare-earth analogs upon fluorine doping [23, 24]. It should be noted that the changes in the lattice parameter due to fluorine doping become more difficult to discern if the ionic radius of the rare-earth is reduced [25]. In fact, no change (or even a small increase) of the cell parameters was observed in superconducting with respect to the parent compound TbFeAsO [26]. This observation can be explained on the basis of the hard-sphere model proposed by Nitsche et al [27].
We also find that the Fe–As–Fe bond angle is approximately 107 in both the parent compound and in the fluorine-doped compound, and hence they lie within the region for which the highest superconducting transition temperatures have been observed in the rare earth compounds upon doping [28], where the distortion of the FeAs4 tetrahedra is small.
Magnetisation data are shown in figure 2(a). There are two clear transitions in the NpFeAsO (T) data. The transition at 25 K in the parent compound is attributed to an NpO2 impurity phase [29], and was not observed in the fluorine-doped sample. The absence of this impurity in the NpFeAsO0.85F0.15 sample can also be observed in our x-ray diffraction data in figure 1. The transition between 55 K and 60 K originates from the antiferromagnetic ordering found previously [15].
Figure 2. The magnetic susceptibility (a) and the specific heat Cp/T (b) are plotted for the parent compound NpFeAsO and fluorine-doped NpFeAsO. Similar experimental data for the parent compound were presented in a previous publication [15]. The color coding of the vertical lines matches the datapoints, and the lines are positioned at the maximum in the temperature derivative of
in (a) and Cp/T in the inset in (b). It can be observed that in both bulk properties, the antiferromagnetic transition temperature is shifted to lower temperatures upon fluorine doping.
Download figure:
Standard image High-resolution imageFigure 2(b) shows the heat capacity data for the parent and fluorine-doped compounds measured in zero field, after correcting for the addenda and for the STYCAST (see Experimental Details). Once again the Néel temperature is very clear, manifesting itself as a sharp feature just below 60 K which coincides with the transition seen in the susceptibility data. The sharp upturn in the specific heat observed at low temperatures is due to a nuclear Schottky anomaly, and is often seen in systems containing neptunium [30]. The small feature observed at 25 K in the parent compound is due to the NpO2 impurity described earlier. Figure 2(b) demonstrates that no superconducting phase transition is observed down to 2 K in the specific heat measurements. In both susceptibility and specific heat measurements it can be observed that the fluorine doping acts to suppress the magnetic ordering temperature, although a quantitative description of the reduction in
is beyond the precision of our measurements.
In figure 3 the room temperature experimental phonon density of states (phDOS) from NpFeAsO (labelled as 'Parent' in the figure) and NpFeAsO0.85F0.15 ('Doped') is plotted. In the inset the raw data is plotted, together with the elastic scattering background that has been subtracted to find the phDOS. Any change in the phDOS as a function of doping is extremely small, and far smaller than those observed in the superconducting NdFeAsO and SmFeAsO families. This result is comparable to that found on the partial Fe phDOS found in the LaFeAsO family [8]. It should be noted that the spectral weight shown above 40 meV arises from multi-phonon scattering processes [22].
Figure 3. (a) The experimental phonon density of states of NpFeAsO and fluorine-doped NpFeAsO are shown in black and red respectively. The inset shows the raw dataset for the parent compound, with the elastic line background shown in blue. (b) The sample holder used in the inelastic x-ray scattering measurements is presented in an exploded view. (c) A photo is shown of the powder samples, sandwiched inside the two single-crystal diamond plates.
Download figure:
Standard image High-resolution imageThere are three main features in the phDOS of NpFeAsO and fluorine-doped NpFeAsO: an intense and broad feature around 10 meV, another peak at about 18 meV and a broad and weak feature with its centre around 33 meV. This three feature structure is consistent with that experimentally determined in other pnictide families [6, 7]. Density functional perturbation theory calculations on LaFeAsO [31] found that the highest energy feature is primarily due to Fe–As phonons, whereas the lower energy features result primarily due to phonons involving the movement of the La atoms (Np in our case). Moreover, measurements of the partial Fe phonon density of states in several rare-earth 1111 pnictides strongly supports these assignments [8]. This understanding helps to explain why the 33 meV feature in the NpFeAsO phDOS has a very similar energy to the corresponding feature in RFeAsO [6–8], as it is derived from the Fe–As layers and somewhat independent of the Np. This is in contrast to the lower energy features in NpFeAsO, which are substantially lower in energy (15% lower) than in all of the rare-earth counterparts. This is due to the significantly larger mass (M) of Np in comparison to the rare-earths, as the energy of a phonon scales with
. This would lead us to expect that the Np phonon energies in NpFeAsO would be about
% of the energy of the rare-earth phonons in RFeAsO, which is in reasonable agreement with our experimental data.
The asymmetric shape of the large broad feature at 10 meV is rather different to the equivalent feature observed in SmFeAsO and NdFeAsO: this is likely due to the strong x-ray scattering cross-section of Np in comparison to the Sm and Nd, which means that the relative intensity of the partial Np phDOS is higher than in RFeAsO. The scattering power scales approximately with Z2, where Z is the proton number, so the relative increase in intensity should be approximately .
4. Conclusions
In summary, NpFeAsO and its fluorine doped derivative show qualitatively the same behaviour, that is, one intrinsic transition, magnetic in origin, occurring between 55 K and 60 K. Previous studies of this transition in the parent compound using neutron scattering [15] and via the 237Np Mössbauer effect [32] have shown that this magnetic transition is entirely due to magnetic ordering of the Np atoms, with an ordered magnetic moment of approximately 1.70 with an ordering wavevector along the c* axis. The observation of one single transition has been supported by both specific heat and transport measurements. Whilst NpFeAsO displays very similar lattice parameters and Fe–As–Fe bond angles to those found to produce the highest superconducting transition temperatures in the rare-earth compounds, we have observed no superconductivity upon fluorine doping [33] in either the specific heat or magnetic susceptibility measurements.10
Replacing oxygen with fluorine slightly lowers the Néel temperature, but the effect is markedly reduced in comparison to the rare-earth analogues. However it should be noted that the symmetry of the magnetic ordering is quite different in NpFeAsO in comparison to the rare-earth pnictides, as the ordering wavevector is along the c* axis, so the underlying physics which governs the reduction in may also be different in NpFeAsO. No evidence for the tetragonal-to-orthorhombic structural transition, which accompanies the SDW transition in the rare-earth 1111 pnictides, was observed using x-ray diffraction in either the parent compound or the fluorine-doped compound down to 5 K. Our study of the phonon density of states provides further evidence that the strong doping-dependent renormalisations of phonon energies observed in NdFeAsO [6] and SmFeAsO [7] are only observed in the superconducting RFeAsO families.
The lack of the tetragonal-to-orthorhombic structural transition in the case of NpFeAsO suggests that the replacement of a localized 4 f shell with an itinerant 5 f shell in the spacer layer between the FeAs layers has disrupted the delicate balance between the nematic order, magnetism and superconductivity found in the superconducting rare-earth pnictides. Moreover, although our work cannot directly further our understanding of the magnetic correlations in the superconducting pnictides, our study raises the possibility that magnetic itinerancy in the spacer layer may suppress the superconducting phase altogether. This is rather different to the understanding proposed for other iron pnictide superconductors with non-magnetic metallic spacer layers [12]. Theoretical understanding of these observations would be of significant interest, as it may help determine which are the important interactions leading to superconductivity in the 1111 family of iron pnictide superconductors.
Acknowledgments
The authors would like to thank D Gambetti for technical assistance during the IXS experiment at ID28 at the ESRF. The authors are very grateful to A Heil, A Hesselschwerdt and F Kinnart for their help in designing and manufacturing the special sample encapsulation used in the experiment at the ESRF.
Footnotes
- 9
It should be noted that in this study oxygen deficiency rather than fluorine doping was used to electronically dope the FeAs layers and drive the system into the superconducting phase.
- 10
We would also like to note here that additional samples of NpFeAsO doped with Co and Mn on the Fe site have also been synthesised, and superconductivity has not been observed in either sample.