Abstract
A recent dynamic model relates the functional near-infrared spectroscopy (fNIRS) measurements to hemodynamic and metabolic parameters. This note reports modified expressions of the new model in terms of cerebral blood volume (CBV), blood flow (CBF) and metabolic rate of oxygen (CMRO2). On the basis of these modified expressions, the new model reproduces known steady state relationships between hemoglobin concentration, CBF and CMRO2, and yields time-dependent relationships that describe transient changes. This new model allows for the translation of the fNIRS measurements into dynamic measures of ΔCBV/CBV0 and the difference ΔCBF/CBF0 – ΔCMRO2/CMRO2|0, provided that some baseline physiological parameters and a relationship between overall, arterial and venous blood volume changes are assumed.
Export citation and abstract BibTeX RIS
1. Introduction
Functional near-infrared spectroscopy (fNIRS) is a powerful tool for non-invasive assessment of cerebral hemodynamics and brain function (Ferrari and Quaresima 2012). fNIRS is directly sensitive to the cerebral concentrations of deoxy-hemoglobin (D) and oxy-hemoglobin (O), which in turn result from the combination and interplay of cerebral blood volume (CBV), cerebral blood flow (CBF) and cerebral metabolic rate of oxygen (CMRO2). As a result, it is highly significant to develop models that can quantitatively relate the fNIRS measurements of D and O to CBV, CBF and CMRO2, so that the fNIRS measurements can be translated into measurements of underlying physiological processes. A number of steady state models aiming at relating asymptotic changes of hemoglobin concentration and functional parameters have been developed (Hoge et al 1999, Mayhew et al 2001, Jones et al 2001) and applied to animal (Jones et al 2001, Dunn et al 2005) and human (Boas et al 2003, Roche-Labarbe et al 2014) studies. A recently introduced hemodynamic model provides analytical, time-dependent expressions that relate D and O (and derived total hemoglobin concentration (T = D + O) and hemoglobin saturation (S = O/T)) to baseline and temporal changes in blood volume, flow velocity and oxygen diffusion rate (Fantini 2014). In this note, I modify the model equations to express them in terms of CBV, CBF and CMRO2. These new expressions are then used to reproduce known steady state relationships reported in the literature and to derive new dynamic relationships that describe transient hemodynamic and metabolic changes. Furthermore, I describe how the new model equations can be used to translate the fNIRS measurements into measurements of ΔCBV/CBV0 and the difference ΔCBF/CBF0 – ΔCMRO2/CMRO2|0.
2. Hemodynamic model
I have recently introduced a new hemodynamic model that relates the time-dependent concentrations of deoxy-hemoglobin [D(t)], oxy-hemoglobin [O(t)] and total hemoglobin [T(t)] in brain tissue to perturbations in the arterial, capillary and venous blood volume [v(a)(t), v(c)(t), v(v)(t), respectively], in the capillary blood flow velocity [f(c)(t)], and in the oxygen diffusion rate constant [] (Fantini 2014):



where ctHb is the concentration of hemoglobin in blood (molHbT/lblood), ϕ is the baseline blood volume, F(c) is the ratio of capillary to large vessel hematocrit (Fåhraeus factor), S is the blood oxygen saturation, α is the rate constant of oxygen diffusion from blood to tissue (s−1), t(c) is the blood transit time in capillaries (s) and the * operator indicates a convolution product. Following the notation convention introduced previously (Fantini 2014), superscripts (a), (c), (v) indicate the arterial, capillary and venous compartments, respectively. The impulse responses associated with the capillary (: RC low pass) and venous (
: time-shifted Gaussian low pass) compartments reflect the low-pass responses related to the blood transit times in the capillaries (t(c)) and venules (t(v)) and are given by (Fantini 2014):


where H(t) is the Heaviside unit step function (H(t) = 0 for t < 0; H(t) = 1 for t ≥ 0).
Here, I intend to rewrite equations (1)–(3) in terms of CBV, CBF and CMRO2, which requires to first determine the relationships between v, f(c), and CBV, CBF, CMRO2.
2.1. Cerebral blood volume
CBV is a measure of blood content in brain tissue and can be defined as the volume of blood per unit mass of cerebral tissue (mlblood/100 gtissue) or per unit volume of cerebral tissue (mlblood/mltissue or %). By considering the latter definition, one can write CBV = T/ctHb, where T has units of molHbT/mltissue and ctHB has units of molHbT/mlblood, so that relative changes of CBV with respect to baseline (CBV0) are given by ΔCBV/CBV0 = ΔT/T0. By using the notation of equations (1)–(3), where ϕ(a), ϕ(c) and ϕ(v) are the baseline arterial, capillary and venous blood volumes (mlblood/mltissue), and v(a)(t), v(c)(t), v(v)(t) are their relative perturbations (%), respectively, one can decompose the CBV into its arterial, capillary and venous components:


so that:

where the last approximate equality follows from equation (3) by considering that F(c)≅1.
2.2. Cerebral blood flow
CBF is a measure of the amount of blood flowing per unit time through a unit mass of brain tissue (mlblood/min/100 gtissue), or through a unit volume of brain tissue (mlblood/min/mltissue). The CBF depends on both the blood transit time (τ) and the blood volume (CBV), according to the following expression:

In the case of cerebral capillary networks, experimental evidence on rats has shown that no capillary recruitment is associated with hypercapnia (Göbel et al 1989, Villringer et al 1994) and with the wake–sleep cycle (Zoccoli et al 1996). In the brain, all capillaries are perfused at any time point, and changes in CBF are directly associated with changes in capillary flow velocity (Kuschinsky and Paulson 1992, Chen et al 1994, Villringer 2012). Therefore, the capillary blood volume, CBV(c), is considered to be a constant. By expressing the capillary blood transit time (t(c)) in terms of the capillary flow velocity (c(c)) and the effective capillary length over the tissue volume of interest (L(c), which is also a constant), one can write equation (9) as follows:

which yields:

where f(c) is the relative change in capillary flow velocity introduced in equations (1) and (2) (Fantini 2014). It is important to observe that t(c) is the physiologically relevant blood transit time, since it is in the capillary bed that the oxygen, carbon dioxide and glucose exchange occurs. From the point of view of the fNIRS measurements, changes in t(c) (i.e. f(c) ≠ 0) induce measurable changes in the tissue concentrations of oxy- and deoxy-hemoglobin, which are propagated through the venous compartments in the volume of interest according to the venous blood transit time t(v) as described by equations (1) and (2).
2.3. Cerebral metabolic rate of oxygen
The CMRO2 is a measure of the amount of oxygen delivered per unit time, per unit mass of brain tissue (mlO2/min/100 gtissue). As done above, one can consider a unit volume (instead of unit mass) of brain tissue, and express the amount of oxygen in moles (instead of milliliters) to result in CMRO2 units of molesO2/min/mltissue. Because the oxygen exchange from blood to tissue only occurs in the capillaries, the CMRO2 can be expressed as the product of the concentration of oxygen molecules in arterial blood (4S(a)ctHb, where the factor of 4 takes into account the four binding sites for oxygen in hemoglobin), the capillary blood volume corrected for the Fåhraeus effect (F(c)CBV(c)), the probability of oxygen release from capillary blood to tissue , and the inverse of the blood transit time in capillaries (1/t(c)):

Because α and t(c) are the only time-varying parameters, one can find the perturbation in CMRO2 by differentiating equation (12) with respect to α and t(c):

where subscripts '0' indicate baseline values. By taking the ratio of equations (13) and (12), and by considering that (see equation (11)) and that
by definition (Fantini 2014), one finds:

Since and
(Fantini 2002), one can finally write:

which relates f(c) and (the relative changes in the capillary flow velocity and in the rate constant for oxygen diffusion) to the relative changes in CBF and CMRO2.
I conclude this section by observing that (Fantini 2002), which is defined as the oxygen extraction factor (OEF), and recalling that CBF = CBV(c)/t(c) (see equation (10)), so that equation (12) can be written as follows:

which yields the following relationship:

2.4. Hemodynamic model expressions in terms of CBV, CBF and CMRO2
On the basis of equations (6), (7) and (15), the hemodynamic model equations [equations (1)–(3)] can be written in terms of CBV, CBF and CMRO2 as follows:
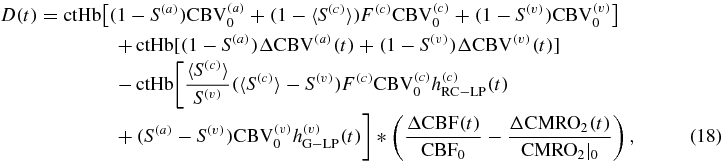
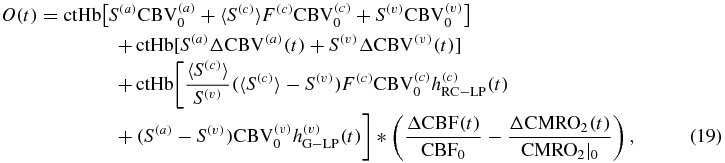

where ΔCBV(c) = 0 to take into account the negligible capillary recruitment and dilation as discussed above (section on CBF). It is worth noting that D(t), O(t) and T(t) in equations (18)–(20) represent absolute concentrations of deoxy-, oxy- and total hemoglobin, respectively, in the brain tissue.
3. Steady state relationships to describe asymptotic changes
The dynamic, time-dependent expressions of equations (18)–(20) yield static, steady state relationships between changes in CBF, CBV and CMRO2, and the corresponding changes in D, O and T, once the impulse response functions are replaced with their integral over the entire time axis (which is equal to 1), and the convolution products are replaced with regular products. The model equations reproduce steady state relationships reported in the literature, and provide analytical expressions for some associated parameters. For example, Hoge et al reported the following expression (Hoge et al 1999):

where is the concentration of deoxy-hemoglobin in venous blood, which is affected by the blood flow velocity in capillaries and by the metabolic rate of oxygen but not by blood volume, so that, using different notation,
where the subscripts
indicate that blood flow and metabolic rate of oxygen are the sources of changes considered. With this notation change, and considering small perturbations, equation (21) is equivalent to:

Equation (22) follows directly from the steady state version of equation (18), under the approximation S(a)≅1, since:

Mayhew et al have further developed equation (23) by expressing the relative change in the concentration of deoxy-hemoglobin in venous blood in terms of the relative change in the tissue concentrations of deoxy-hemoglobin from the venous compartment
, which also includes contributions from blood volume changes (Mayhew et al 2001):

Equation (24) can also be derived by the new model considered here, under the assumption S(a)≅1 (which is typically a good approximation except for pathological conditions involving respiratory or pulmonary oxygen exchange deficits), by recalling that and by observing that, from the steady state version of equations (18) and (20):

Mayhew et al proceeded one step further, by expressing the venous contributions to the tissue concentrations of deoxy- and total hemoglobin (D(v), T(v)) in terms of the measurable overall tissue concentrations of deoxy- and total hemoglobin (D, T) as follows (Mayhew et al 2001, Jones et al 2001):

where γr and γt are constants that take values within the range 0.2–5, with most plausible values around 1 (Mayhew et al 2001). The new model allows one to derive explicit expressions for the coefficients γr and γt by writing equation (26) as follows:

and by imposing the independent equalities of CBF/CMRO2-induced changes and CBV-induced changes, so that:


where I have set (1 − S(a))≅0. By using the reference values of table 2 in Fantini (2014) (namely ,
, S(a) = 0.98, 〈S(c)〉 = 0.74, S(v) = 0.54, F(c) = 0.8), from equations (28) and (29) one finds γr = 0.987 and γt = 2.09ΔCBV(v)/ΔCBV. The parameters γr and γt in equations (28) and (29), while validating equation (26) as given in Mayhew et al (2001), do not coincide with the definitions given in Mayhew et al (2001) since γt in equation (29) takes into account contributions from both capillary and venous compartments (
and
) rather than just the venous compartment, and the ratio ΔD(v)/ΔD used in Mayhew et al (2001) to define γr is not strictly a constant as it depends on ΔCBV(v), ΔCBF/CBF0 and ΔCMRO2/CMRO2|0 (the ratio ΔCBV(v)/ΔCBV in equation (29) is expected to be weakly time dependent, and it is equal to the constant
if
).
4. Dynamic relationships to describe transient changes
The new model yields time-dependent expressions that describe transient changes in cerebral hemodynamics and metabolism. Equations (18)–(20) yield the following time-dependent relationships for the temporal changes of deoxy-, oxy- and total hemoglobin concentrations normalized to their respective baseline values:
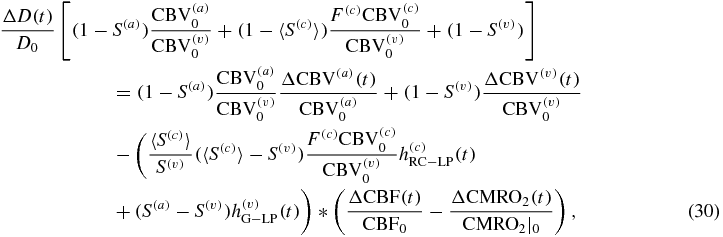
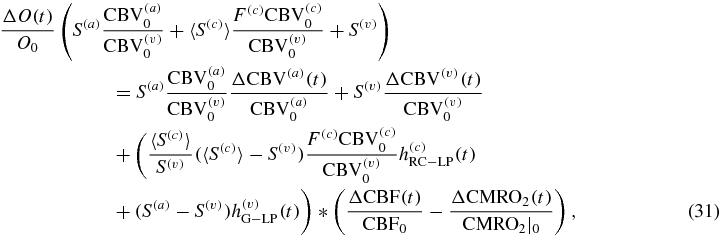

The average capillary saturation (〈S(c)〉) and the venous saturation (S(v)) are related to the arterial saturation (S(a)), the rate constant of oxygen diffusion (α) and the blood transit time in capillaries (t(c)) according to the expressions reported above (see the derivation of equation (15) from equation (14)). The blood transit times in the capillaries and venules (t(c) and t(v), respectively) appear in the impulse response functions; specifically, t(c) is in (see equation (4)) and both t(c) and t(v) are in
(see equation (5)).
5. Discussion
Equations (30)–(32) prompt three major observations:
- (1)in agreement with standard methods in the literature, the relative change of total concentration of hemoglobin in tissue (ΔT/T0) is a direct measure of the relative change in CBV (ΔCBV/CBV0) (see equation (32)).
- (2)
- (3)The fNIRS measurements of the concentrations of the deoxy- and oxy-hemoglobin concentrations (D and O) are affected by the individual arterial and venous blood volume changes, and not just by the overall blood volume changes, which instead completely determine the total hemoglobin concentration changes.
As a result of the above considerations, these are the steps to translate the fNIRS measurements into associated physiological/functional changes according to this new model:
- (I)The relative changes in the CBV (ΔCBV/CBV0) are directly provided by the measured relative changes in the total hemoglobin concentration;
- (II)The following baseline anatomical and physiological parameters need to be assumed (or assessed independently): the rate constant of oxygen diffusion from the microvasculature to tissue (α), the blood transit times in the capillaries (t(c)) and in the venules (t(v)), the relative arterial, capillary and venous blood volume fractions (
,
), and the ratio of capillary to large vessel hematocrit (F(c));
- (III)A relationship between the overall changes in the CBV (ΔCBV/CBV0 = ΔT/T0) and the partial blood volume changes of the arterial and venous compartments (
,
) need to be assumed;
- (IV)
Because the fNIRS, as a standalone technique, is unable to separately measure temporal perturbations in the CBF and metabolic rate of oxygen, an independent measurement of one of them is needed to specify the other from the fNIRS measurements. For example, temporal perturbations in the CBF can be assessed with diffuse correlation spectroscopy (Durduran and Yodh 2014) or a combination of arterial spin labeling and BOLD MRI (Kida et al 2007). Such additional measurements would complement the fNIRS data to generate temporal perturbations of the CBV, CBF and CMRO2 during brain activation.
6. Conclusion
The translation of hemodynamic-based functional imaging data (i.e. fNIRS and fMRI) into underlying physiological/functional processes (CBV, CBF, CMRO2) is of paramount importance for the investigation of the biological mechanisms associated with brain activation. This note has reported modified expressions of a new hemodynamic model (Fantini 2014) to provide analytical relationships between the tissue concentrations of oxy-, deoxy- and total hemoglobin (which are directly measurable with fNIRS), and CBV, CBF, CMRO2 [see equations (18)–(20)]. These analytical relationships yield dynamic expressions that extend previously reported steady state expressions to relate hemoglobin concentration changes to the CBF and CMRO2 changes (see equations (30)–(32)). As detailed in the discussion section, the reported analytical expressions, which contain a limited number of physiologically meaningful parameters (rate constant for oxygen diffusion, blood transit times, baseline blood volume fractions, etc), lead to a straightforward method to translate the fNIRS data into physiological parameters, with the caveat that while CBV/CBV0 can be determined, CBF and CMRO2 cannot be assessed independently but only through the difference ΔCBF/CBF0– ΔCMRO2/CMRO2|0.
Acknowledgments
I thank Angelo Sassaroli and Jana Kainerstorfer for useful discussions. This research is supported by the National Science Foundation (award no. IIS-1065154). The author declares no conflicts of interest.