Abstract
Cyclometalated Ir(III) complexes are attracting extensive attention due to their high emission properties in the visible region. Oxygen sensitive photoluminescence (PL) emissions from single- and multi-layered Ir(III) Langmuir–Blodgett (LB) films are measured. The single-layered LB film on a non-aluminum coated glass substrate shows oxygen sensitive PL emission but its intensity is very weak. In contrast, the multi-layered LB film shows higher intensity but their oxygen sensitivity is lower than that of the single-layered sample. To break the trade-off between intensity and sensitivity, PL characteristics from the LB film on an aluminum-coated glass substrate are investigated. The PL emission from the LB film on the metal layer show higher intensity and good oxygen sensitivity. The mechanism of the emission enhancement is also discussed in this paper.
Export citation and abstract BibTeX RIS
1. Introduction
Luminescent transition metal complexes have been applied as emitting elements for photo-responsive devices.1–13) Among them, cyclometalated Ir(III) complexes are attracting extensive attention due to their high emission properties in the visible region. They are used in optical devices such as photodiodes and oxygen sensors.8–10,13) Their functions are based on the property that energy transfer takes place efficiently from the triplet excited state of an Ir(III) complex to semiconductors or an oxygen molecule in a triplet ground state.13,14)
In our recent work, the hybridization of an amphiphilic Ir(III) complex with a montmorillonite clay was performed by means of themodified Langmuir–Blodgett (LB) method.15–17) According to this method, a floating monolayer of an amphiphilic cationic complex was formed on a subphase containing the exfoliated nanosheets of a clay mineral. Hybridization took place electrostatically between the cationic monolayer and negatively charged clay sheets at an air–water interface.16) The floating hybrid film was deposited onto a solid substrate. The nanometer-thick films had good sensitivity for oxygen gas.17) On the other hand, the challenge for the films is the improvement of the photoluminescence (PL) properties.
Emitted light from LB films on metal nanoparticles or various metallic nanostructures have been studied to improve luminescence performance.18–22) Metal nanoparticles and metallic nanostructures have attracted considerable attention in recent years due to their large optical field enhancements resulting in the strong scattering and absorption of light at surface plasmon resonance.23–27) Plasmon-enhanced fluorescence can be observed from molecules located at a certain distance from plasmonic nanostructures because fluorophore emission quenching occurs in molecules near a metal surface.28) Neal et al. have reported a surface plasmon enhanced emission from dye doped polymer layer on a metal layer. They observed an 11-fold enhancement of light emission using a thin silver layer.29) In this study, luminescence enhancement on an aluminum layer is studied to improve the emission properties of the Ir(III) LB films. The mechanism of the enhancement is also discussed, and then the measured results are compared with the calculation results. We also demonstrate improvement of the signal-to-noise ratio in oxygen sensitive emission from a single layered LB film on an aluminum layer.
2. Experimental procedure
2.1. Sample preparation
We prepared aluminum-evaporated glass substrates where half of the area of the substrate was coated with aluminum but the other half was not coated. A spacer was prepared on the glass substrate by spin coating poly(methyl methacrylate) (PMMA) 2 wt % solution in toluene. The aluminum-evaporated glass substrate with the PMMA spacer was coated with an LB film. The PL enhancement caused by the aluminum layer was evaluated by comparing PL emission from the LB film on the aluminum-coated area with that from the LB film on the non-coated area.
LB films were prepared using an LB trough (USI System FSD-21). A trough with a surface area of 10.0 × 12.0 cm2 was maintained at 20 °C by circulating water. 0.2 mL of chloroform solution containing a perchlorate salt of [Ir(ppy)2(dc9bpy)]+ (denoted PPY) or [Ir(dfppy)2(dc9bpy)]+ (denoted DFPPY) (ca. 5 × 10−5 M) was spread over an aqueous dispersion of a saponite clay (10 mg L−1). After 30 min, the surface was compressed at a rate of 10 cm2 min−1 until the surface pressure reached 10 mN m−1. Keeping the surface pressure at 10 mN m−1 for 30 min, the film was transferred onto the aluminum-coated glass substrate with a PMMA spacer by the vertical deposition method at a dipping rate of 10 mm min−1. Multi-layered films were prepared by the layer-by-layer method. In this procedure, the substrates were dried under vacuum for 30 min after depositing each layer.
2.2. Experimental setup for PL measurement
Figure 1 shows the schematic view of PL measurement setup. A 405 nm laser diode was used as an excitation light source. The sample was irradiated at an angle of 45° using the excitation light. The PL emission from the sample was measured with a CCD spectrometer (StellarNet Bluewave). A dichroic mirror was placed between the sample and the detector of the spectrometer to cut the excitation light.
Fig. 1. A schematic view of PL measurement setup.
Download figure:
Standard image High-resolution image3. Results and discussion
3.1. Oxygen responses of single- and multi-layered LB films
PL emissions from single-layered PPY and DFPPY LB films in the air and vacuum were shown in Figs. 2(a) and 2(b). These samples were fabricated on non-aluminum-coated glass substrates. When measuring the PL spectra, the samples were placed in a vacuum desiccator. The PL spectra from the samples were measured in the air environment, and then the chamber was vacuumed by a pump for 15 min. To investigate the influence of oxygen on the PL emission, we again measured the PL spectra from the samples in the vacuum. The strong peak at 405 nm is caused by the laser diode as an excitation light source. As is evident from Figs. 2(a) and 2(b), the PL emissions of Ir(III) complexes in the air are quenched by oxygen. The oxygen response can be used for an oxygen sensor. However, the PL emissions from the single-layered samples are very weak owing to its thinness. Although long exposure is one of the solutions to the problem of the signal-to-noise ratio, that leads to a slow response time.
Fig. 2. PL emissions from LB films on non-aluminum-coated glass substrates in the air and vacuum: (a) single-layered PPY, (b) single-layered DFPPY, (c) 6-layered PPY, and (d) 10-layered DFPPY.
Download figure:
Standard image High-resolution imageTo increase PL intensity, we also prepared 6-layered PPY and 10-layered DFPPY LB films on non-aluminum-coated glass substrates. Figures 2(c) and 2(d) show the PL spectra of the multi-layered samples in the air and vacuum. The measurement procedure is the same as that for the single-layered LB films. The multi-layered samples show an increase in PL intensity. However, the oxygen sensitivity decreases in the multi-layered samples. Especially, it is difficult to confirm the oxygen sensitivity from the 10-layered DFPPY film. This is because the existence of oxygen influences the PL properties at only the surface of the multi-layered sample. Therefore, the multi-layered LB film is not suitable for oxygen sensing application.
3.2. PL enhancement by a metal layer
PL enhancements by a metal layer or metal nanoparticles are one of the most attractive topics in nanophotonics. We measured the PL emissions from the single-layered PPY LB films with various thickness of PMMA spacers on aluminum-coated glass substrates to achieve high oxygen sensitivity and high signal-to-noise ratio. Before PL measurements, the thicknesses of PMMA spacers were determined by curve fitting of reflection profiles. Reflection spectra were measured by microspectroscopy to obtain accurate thickness. Figure 3(a) shows typical reflection spectra of the single-layered PPY LB films on aluminum-coated glass substrates with different thickness of PMMA spacers. The reflection profile depends on the thickness of aluminum and PMMA layers. To perform curve fitting, reflection spectra were calculated using transfer matrix method.30) In the calculation, the wavelength dispersion of the refractive index of aluminum was taken into account,31) while the refractive index of PMMA was set to be at a constant value of 1.5. The PL emission from the PPY was ignored because the fluorescence excited by halogen lamp was very weak. Figure 3(b) shows the calculated reflection spectra for three sample conditions. In these calculations, we used a reflection spectrum of a glass substrate coated with a 50 nm aluminum layer as a reference spectrum. The calculation parameters are dPMMA = 120 nm and dAl = 50 nm for the blue curve and dPMMA = 120 nm and dAl = 20 nm for the green curve, where dPMMA and dAl are the thicknesses of the PMMA and aluminum layers, respectively. The calculated reflection spectra show interference fringes between the top surface and the aluminum layer. The dips of the blue and green curves appear at 800 nm and their curve profiles are almost the same except for the difference in reflection intensity. This is because the peak and dip wavelengths depend on the thickness of dPMMA. On the other hand, the reflection intensity depends on the thickness of dAl. Since the calculated structure of the green curve has a thinner aluminum layer than that of the blue curve, the green curve shows lower reflection intensity than that of the blue curve. By using the curve fitting method, the thicknesses of the PMMA layers were determined.
Download figure:
Standard image High-resolution imageFig. 3. Typical reflection spectra of single-layered PPY LB films on aluminum-coated glass substrates with a PMMA spacer: (a) measured and (b) calculated results.
Download figure:
Standard image High-resolution imageWe measured PL spectra of samples having different thickness of PMMA layers to investigate the PMMA thickness dependence. Figure 4 shows the PL spectra of the PPY LB films on the different thickness of PMMA layers in the air. Red curves are obtained from the PPY on an aluminum coated area, while black curves are obtained from the PPY on a non-aluminum-coated area. The black curves show almost the same PL properties in spite of the fact that the samples have PMMA layers with different thickness. This is because the refractive index of PMMA is close to that of the glass substrate and the reflection at the boundary between the PMMA and glass substrate is very small. In contrast, the PL spectra on the aluminum layer are quite different in their intensities. The red curves for dPMMA = 62 nm and dPMMA = 108 nm show 9.7- and 2.4-fold increases in PL intensity compared with the black curves at 560 nm, respectively, while these for dPMMA = 117 nm and dPMMA = 130 nm show 0.6- and 0.2-fold decrease, respectively. The PL enhancement by the aluminum layer strongly depends on the thickness of the PMMA spacer.
Fig. 4. PL spectra of the PPY LB films on the different thick PMMA layers: (a) dPMMA = 62 nm, (b) dPMMA = 108 nm, (c) dPMMA = 117 nm, and (d) dPMMA = 130 nm.
Download figure:
Standard image High-resolution image3.3. Mechanism of the PL enhancement
It is well known that metal nanoparticles and metal nano-structures show plasmon-enhanced electric field. However, we here discuss the enhancement by thin film interference effect without the plasmon enhancement. Let us consider light emission from the LB film on the PMMA and aluminum layers. When the emitted light is expressed as A exp(ikPLz − iωt), the reflected light by the aluminum layer can be written by −A exp[ikPL(z + 2dPMMA) − iωt]. Here, A is the amplitude of the emitted light and kPL is its wavenumber for the thickness direction. The amplitude of the interference between the emitted light and its reflected light can be obtained as A[1 − exp(i2kPLdPMMA)]. Since the real part of the amplitude is A[1 − cos(2kPLdPMMA)], the amplitude ratio between the emitted light from the LB film on an aluminum coated area and that from the LB film on a non-aluminum-coated area is given by 1 − cos(2kPLdPMMA).
Interference also occurs when the excitation light enters the PMMA layer. In the same manner, the amplitude ratio between the excitation light entering an aluminum coated area and that entering a non-aluminum-coated area is given by 1 − cos(2kexdPMMA), where kex is the wavenumber of the excitation light for the thickness direction. Therefore, the total enhancement ratio IAl/Iglass taking into account both emitted light and excitation light interference is given by

where IAl is the PL intensity on the aluminum-coated area and Iglass is the that of the non-aluminum-coated area.
Figure 5 shows the enhancement ratio for excitation light, the emission enhancement for emitted light, and the total enhancement ratio. The blue curve represents the enhancement ratio for excitation light with a wavelength of 405 nm expressed as [1 − cos(2kexdPMMA)]2, while the red curve represents the enhancement ratio for emitted light with a wavelength of 560 nm expressed as [1 − cos(2kPLdPMMA)]2. The black curve is the total emission enhancement IAl/Iglass which is the product of the blue and red curves. In this condition, the maximum total enhancement ratio is 14.3 at dPMMA = 78 nm. On the other hand, open circles are the experimentally obtained enhancement ration at 560 nm. The measured results well agree with the theoretical curve based on the interference effect. This indicates that the PL enhancement in our system is caused by interference not plasmon.
Fig. 5. Enhancement ratio for excitation light, the emission enhancement for emitted light, and the total enhancement ratio. Open circles are the experimentally obtained enhancement ration at 560 nm.
Download figure:
Standard image High-resolution image3.4. Oxygen responses of single-layered LB films with an aluminum layer
We investigated oxygen responses of PPY and DFPPY-LB films on aluminum-coated glass substrates. Figure 6(a) shows the PL spectra of the single-layered PPY with a 62 nm PMMA spacer on an aluminum-coated glass substrate in the air and vacuum. Experimental procedures are the same as in Sect. 3.1. Compared with the spectra shown in Fig. 2(a), the signal-to-noise ratio is clearly improved while the oxygen sensitivity is maintained. Figure 6(b) shows the PL spectra of the single-layered DFPPY with a 235 nm PMMA spacer on an aluminum-coated glass substrate in the air and vacuum. We also confirmed that the single-layered DFPPY has good oxygen sensitivity.
Fig. 6. PL spectra of the single-layered LB films on the aluminum layer in the air and vacuum: (a) PPY and (b) DFPPY.
Download figure:
Standard image High-resolution imageThe signal-to-noise ratio S/N can be defined as
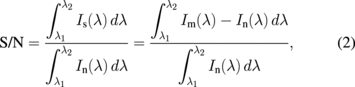
where Is an In are the PL intensities of the signal and noise, respectively. λ is the wavelength of the PL light, and Im is the experimentally measured PL intensity which is the sum of Is and In. That is, Is can be obtained from Im − In. In this study, we use the values of In by averaging 10 sets of noise data obtained from the CCD spectrometer in the dark condition. We calculated the S/N values of the spectra in Figs. 2 and 6. The S/N values for PPY and DFPPY are summarized in Table I. The values for PPY were integrated from 500 to 700 nm, while the values for DFPPY were integrated from 450 to 600 nm. The aluminum layer improved the S/N characteristics for PPY and DFPPY approximately 10 and 8 times, respectively.
Table I. Values of signal-to-noise ratio S/N for four samples in the vacuum and air.
Environment | S/N | |||
---|---|---|---|---|
PPY | PPY/PMMA (62 nm)/Al | DFPPY | DFPPY/PMMA (235 nm)/Al | |
Vacuum | 9.1 | 86.6 | 9.4 | 76.4 |
Air | 6.2 | 66.0 | 6.1 | 45.4 |
4. Conclusions
Oxygen sensitive PL emissions from the PPY and DFPPY LB films were investigated. The single-layered LB films on the non-aluminum-coated glass substrates showed oxygen sensitive PL emission but their intensities were very weak. In contrast, the multi-layered LB films had higher intensity but the films show low oxygen sensitivity. This was because emission quenching occurred only near the surface of the LB films. We investigated PL characteristics from the PPY and DFPPY LB films on the aluminum-coated glass substrates to break the trade-off between intensity and sensitivity. The PL emission from the PPY LB film depended on the PMMA spacer thickness. We observed the 9.7-fold PL enhancement from the PPY on the aluminum coated glass with the 62 nm PMMA spacer. The PMMA thickness dependence of the enhancement ratio well agreed with the calculated result based on interference. We measured oxygen responses of the PPY and DFPPY-LB films on aluminum-coated glass substrates. The PL spectra of the single-layered PPY and DFPPY films on aluminum-coated glass substrates in the air and vacuum showed good oxygen sensitivity. Compared with the spectra without a metal layer, the signal-to-noise ratio was clearly improved while the oxygen sensitivity was maintained.
Acknowledgments
This work was supported by JSPS KAKENHI Grant Numbers 15K05988 and 17H03044.