Abstract
Recent studies of nuclei far from the stability line have revealed drastic changes in nuclear orbitals and reported the appearance of new magic numbers and the disappearance of magic numbers observed at the stability line. One of the important reasons for such changes is considered to be because of the effect of tensor forces on nuclear structure. Although the role of tensor forces in binding very light nuclei such as deuterons and 4He has been known, direct experimental evidence for the effect on nuclear structure is scarce. In this paper, I review known effects of tensor forces in nuclei and then discuss the recently raised question of s–p wave mixing in a halo nucleus of 11Li. Following these reviews, the development of a new experiment to see the high-momentum components due to the tensor forces is discussed and some of the new data are presented.
Export citation and abstract BibTeX RIS
1. Pions, tensor forces and the binding of light nuclei
The pion exchange interaction includes the central force and the tensor force with the same strength. A recent ab-initio-type calculation using the Green-function Monte–Carlo method (GFMC) by an Argonne group showed that the tensor interactions as well as three-body interactions are particularly important for the binding of light nuclei [1]. The calculation also shows that 80% of the potential energy is from the pion exchange, i.e.
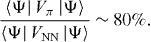
The importance of the tensor interactions can be seen in the binding of deuterons as shown in table 1 [2]. Among the potential energies, central interactions give only 4.46 MeV but tensor interactions give −16.64 MeV. Other contributions such as the LS interaction are also small compared with the tensor interactions. This strong binding is from the cross term of the S-wave and D-wave in spite of a small D-wave amplitude in the wave function, 5.78%. It is seen that the spatial extension of the D-wave is much smaller. This is due to the fact that the tensor interaction does not have a hard core as shown in figure 1. The final wave functions are shown in figure 2. The D-wave has a peak at a shorter distance from the center and indicates a larger contribution of the high-momentum part of the wave function.
Figure 1. The shape of potentials for pion exchange interactions.
Download figure:
Standard imageFigure 2. The S- and D-wave functions in deuterons.
Download figure:
Standard imageTable 1. Binding of deuterons by AV18 interaction.
Energy | −2.24 MeV |
---|---|
Kinetic | 19.88 |
(SS) | 11.31 |
(DD) | 8.57 |
Central | −4.46 |
(SS) | −3.96 |
(DD) | −0.50 |
Tensor | −16.64 |
(SD) | −18.93 |
(DD) | 2.29 |
LS | −1.02 |
P(D) | 5.78% |
Radius | 1.96fm |
(SS) | 2.00fm |
(DD) | 1.22fm |
A similar situation is seen in 4He binding as seen in table 2. Although the mixing amplitude of D-wave is 12.8%, the potential energy due to the tensor interaction is ∼70 MeV and provides almost the same binding energy as that from the central interaction. The main part of the D-wave mixing can be expressed as due to two-particle two-hole excitations under the selection rule of the tensor interaction, ΔL = 2 and ΔS = 2 as shown in figure 3. This excitation goes up to very-high-energy orbitals (if we use shell model base wave functions) and thus includes high-momentum components in the wave function. It is important to note that the large part of the binding energy comes from the tensor interaction that has a shorter range and thus the contribution from high-momentum part of the wave function is important.
Figure 3. 2p–2h excitation due to the tensor interactions.
Download figure:
Standard imageTable 2. Binding of 4He. The calculations were performed by Sakai et al [19].
![]() |
The tensor interaction by the pion exchange interaction VπT is expressed as

where mπ is the pion mass, q is the momentum transfer and S12 is the tensor operator,

Under one-pion exchange, the momentum transfer is (P'1 − P1). If nucleon wave functions are of mean field type, the momentum space covered by nucleons is restricted and thus q cannot be very large. The largest transferred momentum is only available for the exchange term, that is, J'1 = J2. The potential energy is still small because of the restricted high-momentum component in the wave function (see figure 4).
Figure 4. Pion exchange and tensor interactions. Ji expresses the spin and Pi the momentum of nucleon.
Download figure:
Standard imageWhen two-pion exchange is included, the intermediate states include two-particle excitation and the momentum of the nucleon in the intermediate state is not restricted and can thus include very-high-momentum components and gain in binding energy. A mean field model of a nucleus is modeled by one-particle exchange interactions and thus cannot include the large gain in binding energy by high-momentum components.
2. Importance of tensor forces in nuclei
In addition to the binding of nuclei, the importance of tensor forces in nuclei has been discussed from several different viewpoints. One of the recent findings is related to the changes in magic numbers. Studies of nuclei far from the stability line showed the change in magic numbers, disappearance of the traditional magic numbers (8, 20, 28,...) and appearance of new magic numbers (16, ...) [3, 4]. Otsuka et al [5] have explained those changes of magic numbers by the change of single-particle orbitals by the tensor interactions. The basic reason for this effect is explained by figure 5. The figure shows orbitals of protons and neutrons for neutron-rich nuclei. The major shells for protons and neutrons are often different in neutron-rich nuclei as shown in the figure. Here j = l + 1/2 orbitals are expressed as j> and j = l − 1/2 orbitals are expressed as j<. The tensor interaction is attractive between j'> and j< orbitals and repulsive between j'> and j> orbitals. The monopole interaction that gives the binding energy is proportional to the number of particles in an orbital. The j> orbital is less and less bound when the number of neutrons in the j'> orbital increases. On the other hand, the j< orbital is more bound for a larger number of neutrons in the j'> orbital. The energy gap between j> and j< orbitals, therefore, changes if the number of neutrons changes, providing a mechanism of changing spacing between single-particle orbitals.
Figure 5. Effects of tensor interactions for neutron-rich nuclei.
Download figure:
Standard imageThis effect of the tensor force is described in more detail by Otsuka et al [5]; therefore, in this paper, I will not go into the details of the discussion.
2.1. Magnetic moments of mirror nuclei and 〈σ〉
Magnetic moments of nuclei provide unique information on the details of configuration mixing in nuclear structure. One of the most important is the fact that the operator of a magnetic moment does not include the radial operator and thus is only sensitive to the angular momentum part of a wave function except for the total normalization of a wave function. A detailed study of the magnetic moments has been performed for mirror nuclei.
The magnetic moments of mirror pairs in p and sd shells have been analyzed in detail for T = 1/2 nuclei. The expectation values of the isoscalar spin and orbital angular momentum, 〈σ〉, 〈l〉, and the isovector spin and orbital angular momentum, 〈τσ〉, 〈τl〉, respectively, have been obtained and the effects of configuration mixing were shown clearly. An isoscalar and an isovector magnetic moment (μs and μv) of a mirror pair (T> and T<) are related to the expectation values as

where gl is the orbital g factor and μ are nucleon magnetic moments and

for free nucleons glp = 1, gln = 0, μp = 2.792 and μN = −1.913.
By using and equation (3), one can obtain the isoscalar expectation values. The isovector expectation values can be obtained if one combines the 〈τσ〉 values with the beta-decay ft-value between mirror states.
An important finding here is that most of the expectation values are in between the two single-particle values for j = l + 1/2 and j = l − 1/2 orbitals as shown in figure 6. The linear dependence of the expectation values on the number of valence nucleons reflects the first-order configuration mixing. The expectation values should be equal to the single-particle values for the mirror pairs of doubly closed shell ± 1 nuclei, because the first-order configuration mixing does not affect the expectation values. However, the experimental values for such nuclei deviate from the single-particle values significantly as shown in table 3 for pairs of A = 15, 17, 39 and 41.
Figure 6. Spin expectation values of the mirror nuclei.
Download figure:
Standard imageTable 3. Expectation values of the isoscalar spin of mirror nuclei.
A | Orbital | δ〈σ〉/exp〈σ〉 (%) | δ〈σ〉/〈σ〉 (HJ) (%) |
---|---|---|---|
15 | p1/2 | −49.4 | −63 |
17 | d5/2 | −13.6 | −18 |
39 | d3/2 | −61.9 | −55 |
41 | f7/2 | −11.9 | −19 |
Some important considerations are given below.
- 1.If a nucleon–nucleon (n–n) interaction is central, the total spin is a constant of motion.
- 2.The expectation values of spin in nuclei with an LS-closed shell ± 1 nucleon are then the same as their Schmidt values, irrespective of the degree of configuration mixing.
- 3.In order to modify the values, one has to have non-central interaction.
- 4.Pion exchange potential includes a strong tensor force.
Theoretical calculations including the tensor forces have been performed for the second-order effects including high-excitation energy [6]. They could reproduce the experimental values with several interactions such as the Hamada–Johnston (HJ) and Paris potentials. Reasonable agreement was seen in all cases when the mixing up to 12ℏω excitations was included in the calculations. Examples of the results for the HJ potential are shown in table 3.
The theoretical conclusions for the spin expectation values are summarized as follows [7]:
- 1.First-order configuration mixing is very important.
- 2.The Δ-hole excitation does not contribute in first-order perturbation to the isoscalar magnetic moment.
- 3.The contribution from meson exchange currents and second-order Δ-hole excitation is negligibly small.
- 4.Thus, the quenching of the
for a good single-particle state provides the best evidence for the second-order configuration mixing, mainly due to the tensor force.
2.2. s1/2 and p1/2 mixing in 11Li halo
One of the most important questions in halo nuclei is the mixing of (1s1/2)2 and (0p1/2)2 wave functions in the two-neutron halo of 11Li.
Such evidence has been obtained from several different observations. Figure 7 presents the transverse momentum distribution of 10Li fragments obtained by summing the final state 9Li momentum (p9Li) and the forward neutron momentum (pn) measured by Simon et al [8]. That is, under the assumption discussed above, the momentum distribution of the 10Li fragment is produced by one neutron removal from 11Li. They concluded that the neutron wave function of ground state 11Li includes two components with different parity single-particle wave functions as

where two neutrons in s and p orbitals are mixed with almost equal amplitude. Although other components such as (1p3/2)2 may be included, its contribution was suggested to be small from the analysis of the angular correlations shown below.
Figure 7. Transverse momentum (px) distribution of 10Li, reconstructed from the momenta of 9Li and the neutron measured in coincidence. Dashed and dotted lines represent (1s1/2)2 and (0p1/2)2 single-particle momentum distributions calculated for one of the halo neutrons in 11Li, including core shadowing and the experimental resolution. The solid line represents the best fit to the data obtained with a 45% (1s1/2)2 contribution. Adapted from [8].
Download figure:
Standard imageThe mixing of different parity single-particle states is also observed in the angular correlation of the fragments. Figure 8 shows the distribution of the angles (θnf) between the direction of the 10Li momentum and the direction of the relative coordinate of 9Li and the neutron. The forward–backward asymmetry, inclusion of odd terms in cos(θnf), clearly indicates the mixing of different parity states. The observed strong asymmetry in the angular correlation function gives a direct and model-independent indication of the mixing of states with different parities (0p1/2 and 1s1/2) in the ground state of 11Li. The contribution of the (2s1/2)2 state to the 11Li ground-state wave function was determined to be (45 ± 10)%.
Figure 8. Distribution of the decay neutrons from 10Li formed in 11Li neutron knockout reactions. The inset shows a schematic diagram of the reaction where θnf is the angle between the momentum direction of 10Li and the direction of the n + 9Li relative momentum pnf . The distribution asymmetry can be explained only if one assumes contributions from interfering s and p states in 10Li. Adapted from [8].
Download figure:
Standard imageThe mixing of s- and p-waves was also observed in the two-neutron transfer reaction at low energy. The experiment was done at the ISAC-2 facility at TRIUMF that provides a high-intensity 11Li beam up to 55 MeV [9]. The measurement was made using an active target MAYA [10] that enabled us to detect the low-energy recoil tritons. The angular distribution of 11Li(p, t)9Li reactions determined for 36.9 MeV incident 11Li is shown in figure 9. Theoretical calculations were performed including one-step as well as two-step sequential transfer processes via 10Li [9]. The curves in figure 9 show the results of the calculations. The wave function (p1/2)2 with no n–n correlation gives very small cross sections (dashed curve) that are far from the measured values. Also the P0 wave function, with n–n correlation but with a small (s1/2)2 mixing amplitude, gives too small cross sections. The results of the P2 (31% s1/2) and P3 (45% s1/2) wave functions, which include the correlation and large mixing of (s1/2)2, fit the forward angle data reasonably well but the fitting near the minimum of the cross section is unsatisfactory. Although the calculations do not show perfect agreement with the entire angular distribution data, a comparison of the magnitude of the data and the theory indicates the importance of the mixing of (p1/2)2 and (s1/2)2 waves in addition to the strong correlation between two halo neutrons and the core.
Figure 9. Differential cross sections of 11Li(p, t)9Li reaction to the ground state of 9Li. Theoretical predictions are shown by curves. See the text for the difference in the wave function.
Download figure:
Standard imageSuch a mixing of s and p waves in 11Li halo has also been observed in the beta-decay measurement and gave a 30–50% contribution from s1/2 that is consistent with the reaction measurement shown above [11].
Although an equal mixing of 1s1/2 and 0p1/2 waves is observed in experiments, it is not explained by the theoretical models. Recently, a new model that account for the tensor forces was developed as the tensor-optimized shell model (TOSM) [12]. This model explicitly includes the 2p–2h space in the calculations to include tensor interactions. The size parameters of each orbitals are treated as variational parameters to obtain a fast convergence of the energy. This model reproduces the binding of 4He very well, showing the fast convergence. Examples of the results using AV18 interaction are shown in figure 10 for the Li isotopes. The excitation spectra of Li isotopes are well reproduced.
Figure 10. The results of TOSM for Li isotopes.
Download figure:
Standard imageBased on this model, the mixing of s- and p-waves is successfully explained as Pauli blocking effects by the tensor interactions and pairing interactions. Under this model, as shown in figure 11, the ground state of 9Li includes configurations denoted as 'Pairing' and 'Tensor' in addition to 0p–0h configurations. The 'pairing' configuration has a large two-neutron amplitude in 1p1/2 orbital. The 'tensor' configuration has a proton and a neutron in 1p1/2 orbital excited from 1s1/2 orbital. It is due to the selection rule of the tensor interaction, ΔL = 2, ΔS = 2.
Figure 11. Schematic illustration of the Pauli blocking in 11Li.
Download figure:
Standard imageFor forming 11Li, two possibilities can be considered, one is to put two neutrons in the 1p1/2 orbital (1p)2 and the other is to put them in the 2s1/2 orbital (1s)2. It is not possible to put two neutrons in the 1p1/2 orbital for 'pairing' or 'tensor' configurations because those orbitals have already been occupied. This means that the energy gain from pairing and tensor interactions existing in 9Li are lost in this configuration. On the other hand, two neutrons can be in 2s1/2 orbitals without disturbing 'pairing' and 'tensor' configurations and thus gaining energy through those interactions. It should be noted that it is not necessary that the single-particle orbitals 2s1/2 and 1p1/2 are very close to each other. Although 2s1/2 orbital is still higher than 1p1/2 orbital, finally the (1p)2 and (1s)2 configurations are very close in energy and thus the mixing occurs. The results of TOSM calculations are shown in figure 12. Good agreement with the experimental values of the mixing amplitude P(s2) and the matter radius is obtained. Other observables such as nuclear radii and moments were also explained well.
Figure 12. (1s)2 probability P(s2) and matter radius Rm of 11Li together with the experimental data. (a) [8], (b) [9] and (c) [13]. 'Present' shows the final result of the calculations [12].
Download figure:
Standard imageThis mechanism of configuration mixing, in particular the 2p–2h excitation, depends on the available configurations in the region of a nucleus. Therefore, a sudden change of nuclear character may appear at certain proton numbers or neutron numbers. Further studies are necessary to see such effects on heavier halo nuclei. The other effects of the tensor forces are an important subject for future work.
3. Momentum distribution of nucleons in nuclei
3.1. Theoretical predictions
As already mentioned several times above, high-momentum components of nucleons in nuclei play an important role in gaining the binding energy. What do we expect the effect of tensor forces in the momentum distribution to be? Figure 13 explains the relation between the momentum distribution and the physics origins. The most important origin of the momentum distribution is the movement of nucleons in a nuclear potential. Although the detailed momentum distributions depend on an orbital of nucleons, the main feature of the momentum distribution is determined by the size of the potential as typically expressed by Fermi momentum. Thus it mainly has momentum below 1 fm−1 as shown by the red line in figure 13. The momentum distributions are also affected by the n–n correlations, in particular at short distances. One of the well-known origins is the short-range repulsion of the central forces. The characteristic momentum is determined by the range and sharpness of the rise of the short-range repulsion. The rough sketch of the momentum distribution by this origin is shown by the green curve in the figure. The tensor forces also give a characteristic range as shown in the n–n interaction potential (top left panel in figure 13) and make a large contribution at momentum around 2 fm−1 as shown in figure 13 by the blue curve.
Figure 13. Origins of the momentum components of nucleons in a nucleus.
Download figure:
Standard imagePredictions of the momentum distributions with tensor interactions have been made by several theoretical models, such as GFMC [14], UCOM [15] and the three-body cluster model [16]. Figure 14 presents the results of the calculations. For all the models, an enhancement of the momentum density at around 2 fm−1 due to the tensor force is seen. In GFMC calculations, the momentum density is enhanced by two to three orders of magnitude when the AV18 potential that includes the tensor force is used. In UCOM, the range parameter of the tensor force is not uniquely determined, and thus several range parameters were assumed, as shown by α, β and γ. The amount of enhancement strongly depends on the parameter but again the enhancement is seen at ∼2 fm−1. The three-body multi-cluster model shows an interesting difference in the momentum distribution between 6He and 6Li. The momentum distributions are shown for the valence nucleons in figure 14(3). The momentum distribution for 6Li presents an enhancement of momentum density by two orders of magnitude compared with that of 6He at ∼2 fm−1. Because of the selection rules of the tensor interaction, an excitation of the nucleon pair occurs only for a deuteron-like object and thus like nucleon pairs cannot be excited. It is for this reason that 6He, in which the two valence nucleons are both neutrons, the momentum density at ∼2 fm−1 is much lower than that of 6Li. The observation of such a difference in momentum distribution is direct evidence of the effect of tensor interaction on the high-momentum component. A high-intensity 6He beam is necessary for this experiment but GSI/FRS may be the best place for this experiment.
Figure 14. Momentum distributions of nucleons inside nuclei by using (1) GFMC, (2) UCOM and (3) the three-body multi-cluster model. All of them show an enhancement in magnitude of the momentum distribution at about 2 fm−1.
Download figure:
Standard image3.2. Recent experimental developments
To observe the momentum distribution of nucleons in a nucleus, quasi-free knockout reactions (p, 2p) or (e, e'p) measurement may be considered. Although they are a powerful tool to see the distribution at small momenta, they are not the best tool to see the high-momentum component that is orders of magnitude smaller in amplitude. The reason for this is briefly explained in figure 15. When knocked out protons are observed, they form a strong peak at two-body kinematics between the incident particle and the kicked out proton that had an internal momentum equal to ∼0, where the momentum density is maximum. The high-momentum part of interest has density that is four orders of magnitude smaller. Therefore, any complication apart from the pure quasi-free process, such as final state interactions and multiple scatterings, makes it difficult to observe the original momentum amplitude if they affect more than 10–4 in the spectrum. It is extremely difficult, if not impossible, to determine such effects with an accuracy of 10–4.
Figure 15. The observation of momentum distribution by (p, 2p) and (e, e'p) reactions. A peak of the observed momentum distribution is from zero-momentum nucleons.
Download figure:
Standard imageA possible method that is insensitive to the low-momentum component is by a nucleon pick-up reaction such as (p, d) or (p, dp) reactions. The reason is as follows.
In pd elastic scattering, a strong increase in the differential cross section is observed at backward angles (see figure 16). This increase is understood as an exchange reaction of neutrons. A high-momentum neutron in a target deuteron is picked up by an incident proton to form a deuteron in the final state. The transferred momentum PF is exactly the internal momentum of the neutron in the initial state. The cross section σF under the Born approximation is written as
Figure 16. A pd elastic scattering cross section at 135 MeV. The backward increase is due to the neutron pick-up reaction.
Download figure:
Standard image
where K is a phase space constant, BD is the deuteron binding energy and M is the nucleon mass. The deuteron wave function is expressed by φ(r). The last term of the equation indicates that the cross section is proportional to the amplitude of the nucleon with an internal momentum exactly the same as the transferred momentum [17].
A (p, d) reaction at forward angle (the deuteron at small scattering angles) occurs with exactly the same mechanism. The incident proton picks up a neutron in the target with internal momentum exactly the same as the transferred momentum. The cross section is proportional to the amplitude of the momentum distribution with that momentum. Therefore, it is most suitable to see the momentum distributions by changing the transferred momentum. It is desirable to perform the experiment at 0° scattering angle so that the complication due to reaction mechanisms is at minimum.
Another possibility is quasi-free (p, pd) and (p, nd) reaction at forward deuteron emission. Because a high-momentum neutron is expected to be correlated with another nucleon, this nucleon may be emitted to the backward direction just like the proton emitted in the backward angle in a pd elastic scattering. This proton carries also the momentum as an internal momentum. The (p, pd) reaction picks up a neutron from a correlated pair of a proton and a neutron and thus is sensitive to the tensor interaction. On the other hand, the (p, nd) reaction picks up a neutron from a correlated pair of two neutrons and is sensitive only to the short-range correlation due to the central repulsive core. Therefore, the comparison of those reactions may provide unique information on the effect of tensor interactions.
The first measurement aiming at observing the effect of tensor interactions was made at the Ring Cyclotron facility, RCNP, Osaka University using 16O(p, d)15O reactions at 200–400 MeV incident protons. As noted above it is desirable to use 0° scattering angle for this measurement but the available energy at RCNP is limited to 400 MeV and thus a momentum transfer of 2 fm−1 (400 MeV c−1) could not be reached at 0°. To obtain the momentum transfer of 400 MeV c−1 at 0°, much higher energies of incident beams are necessary as shown in figure 17. In the experiment a scattering angle of 10° was used to obtain a momentum transfer of 400 MeV c−1 at 400 MeV incident energy.
Figure 17. Momentum transfer for the (p, d) and (p, pd) reactions at high energy.
Download figure:
Standard imageThe Grand Raiden spectrometer was used for the detection of deuterons from ice (H2O) targets of 30 − 62 mg cm−2. Incident beam energies were Ep = 198. 295 and 392 MeV. The obtained spectra for Ep = 198 and 392 MeV are shown in figure 18. As expected the ground state of 15O (1/2−) and the lowest 3/2− excited state are produced by large cross sections. Those states are produced by picking up a neutron either from p1/2 or p3/2 orbitals.
Figure 18. The excitation spectra of the 16O(p, d)15O reaction at various incident energies.
Download figure:
Standard imageAn interesting observation is a very strong transition to the doublet of positive parity states (1/2+, 5/2+). This peak is even higher than that of the ground-state transition at 392 MeV. With a view of the usual pick-up reaction it is not possible to excite the positive parity state with a one-step reaction from 16O. What is the mechanism of excitation of positive parity states?
An excitation spectrum at a lower incident beam energy (45.34 MeV) is shown in figure 18(c) for comparison. It is clear that the excitation of those positive parity states is much weaker at a low energy.
Therefore, the cross section of the positive parity state has a much stronger energy dependence. The difference of the cross sections is presented in figure 19 by the ratio of this cross section to that of the ground-state transition. R+ is the ratio of the cross section between the positive parity state and the ground state and R− is the ratio between the 3/2− state and the ground state. It is clearly seen that the fact that the relative cross section to the positive parity state increases by more than 30 times at high energies show a steep contrast to R−.
Figure 19. The ratio of the cross sections to different states. R+ is the ratio of the cross section between the positive parity state and the ground state and R− is the ratio between the 3/2− state and the ground state. Dashed and dotted curves are the results of CDCC-BA calculations with the usual shell model wave function. The blue curve is a guide to the eyes.
Download figure:
Standard imageIn general, the cross sections of all states diminish with increasing proton beam energy, i.e. increasing momentum transfer. This trend is particularly pronounced for the ground state, which is evidence of quickly diminishing high-momentum neutrons in the initial ground-state configurations. The relative increase of the positive-parity states at very large momentum transfer apparently indicates a slower decrease or a relative increase of the high-momentum component compared with the negative-parity states.
We examine the mechanism of exciting the positive-parity states. If we assume that the 16O wave function is pure doubly closed, then the positive-parity state cannot be excited by a single step process. However, the multi-step process cannot be the main reason for the excitation because a relatively larger cross section is observed. The multi-step process is considered to be less probable at higher beam energies where the nucleon–nucleon cross sections are much smaller than that at lower energies.
Tensor interactions make 2p–2h excitations and mix new configurations in the 16O ground state as shown in figure 20. The pickup of a neutron in the sd shell, in a mixed configuration, can be a production mechanism of the observed positive parity state. The mixing amplitude is small and probably about 10% if we imagine from the D-wave mixing in 4He nuclei. However, this wave function has large high-momentum components because of the tensor interaction.
Figure 20. Configuration of 16O including the effect of tensor interactions.
Download figure:
Standard imageThe effect of the tensor interaction is small or negligible when a neutron is picked from p-shells, but it dominates when a pick-up occurred from the sd shell that is mixed in the wave function by tensor interactions. The ratio of the cross section R+, therefore, increases as the transferred momentum becomes larger. The strong transition to positive parity states in 15O from (d, p) reaction, therefore, suggests an enhancement of the momentum distribution at larger momentum due to the tensor interactions.
In summary, we have performed an experiment at RCNP, Osaka University using the 16O(p, d) reactions with proton beams at 198, 295 and 392 MeV to search for direct evidence on the effect of the tensor interactions in light nuclei. Differential cross sections of the one-neutron transfer reactions populating the ground states as well as several low-lying excited states in 15O were measured. By considering the ratio of the cross section for each excited state and that for the ground state over a wide range of momentum transfer, we have observed a marked enhancement of the ratio for the 1/2+ and/or 5/2+ state(s) in 15O. The result indicates a relative increase of high-momentum neutrons in the initial ground-state configuration with neutron(s) in 1s1/2and/or 1d5/2 orbitals, and thus may be a direct signature of the effect of the tensor interactions in 16O. This work shows that one-nucleon transfer reactions, e.g. the (p, d) reaction, afford a useful means of probing the effect of the tensor interactions in nuclei.
Acknowledgments
Part of this work was supported by the Grant-in-Aid program of the Japanese Government. The author gratefully acknowledges support from the MEXT of Japan and from the People's Republic of China Government and Beihang University under the Thousand Peoples Plan. He also thanks Dr A Tosaki for his support of the Cosmonuclear Physics Group of Osaka University.