Abstract
This article analyzed the fusion dynamics of stable nuclei, loosely bound nuclei and halo nuclei within the view of coupled channel approach and the energy dependent Woods–Saxon potential model (EDWSP model). The different projectiles ( and
are bombarded onto common spherical target isotope (
wherein target isotope exhibits inelastic surface excitations as dominant mode of coupling. The fusion dynamics of
reactions, wherein there is no signature of fusion suppression at above barrier energies, are adequately explained by both theoretical approaches in domain of Coulomb barrier. In case of
reactions, the couplings of inelastic surface excitations of colliding systems remove the discrepancies between below barrier fusion data and expectations of one-dimensional barrier penetration model. However, at above barrier energies, the fusion excitation function data are suppressed in comparison to the theoretical predictions of both approaches. Interestingly, this suppression of above barrier fusion data is minimized by the present model calculation by a factor of 7% with respect to a value reported in literature. Within the view of the EDWSP model calculations, the above barrier fusion data of
reaction are hindered by a factor of 25% and by a factor of 17% for
reaction which is smaller than a value as pointed out in literature. The observed sub-barrier fusion dynamics of
and
reactions are reasonably described by the coupled channel model and the EDWSP model. Furthermore, the applicability of the EDWSP model has been tested for exploration of the fusion of
reaction and hence due to barrier modification effects, the EDWSP model successfully explains the observed fusion enhancement of
reaction. The present calculations clearly indicate that different theoretical approaches (coupled channel formulation and EDWSP model) induce similar kinds of barrier modification effects in the heavy ion fusion reactions.
Export citation and abstract BibTeX RIS
1. Introduction
The fusion dynamics of stable nuclei, light loosely bound nuclei (weakly bound nuclei) and/or halo nuclei at near and sub-barrier energies has received attention in the last few decades. It has been well established in literature that the heavy ion fusion cross-section in the close vicinity of the Coulomb barrier are strongly influenced by the nuclear structure degrees of freedom of collision partners. In the fusion of tightly bound nuclei, the coupling between relative motion of colliding nuclei and their intrinsic degrees of freedom such as inelastic surface excitations (spherical nuclei), rotational states (deformed nuclei) and nucleon (multi-nucleon) transfer channels leads to the substantially large sub-barrier fusion enhancement over the expectations of one-dimensional barrier penetration model [1–4]. However, in the fusion of loosely (weakly bound or halo nuclei) bound nuclei; the probability of breakup channel strongly influences the mechanism of fusion process. Furthermore, theoretical calculations due to Hussein et al [5, 6] suggested that the fusion excitation functions are hindered because of breakup effects while theoretical calculations due to Dasso et al [7, 8] suggested that the fusion cross-section is enhanced due to breakup effects. For instance, the fusion of [9],
[10, 11] and
reactions [12] claim sub-barrier fusion enhancement while in the fusion of
[13],
[14],
[15] and
reactions [16, 17], a large fusion hindrance at above barrier energies was observed. This idea leads to the exploration of a number of reactions involving weakly bound colliding systems but the enhancement or suppression of fusion cross-section due to breakup effects is still at a controversial stage and further investigations are needed for its clarifications. In the fusion of weakly bound nuclei, one can believe that the breakup process has strong impacts on the energy dependence of low energy fusion cross-sections [9–22]. In this regard, the present article analyzed the fusion of
and
reactions [10, 11, 23–36] within the view of the energy dependent Woods–Saxon potential model (EDWSP model) and coupled channel approach.
Since, the channel coupling effects strongly affect the magnitude of fusion cross-sections across the Coulomb barrier; therefore, the theoretical calculations are performed by using the EDWSP model [37, 38] along with one-dimensional Wong formula [39] and coupled channel code CCFULL [40]. The role of inelastic surface vibrations of colliding pairs is entertained within the context of the coupled channel calculations performed by using the code CCFULL wherein the static Woods–Saxon potential has been employed for addressing the influence of intrinsic degrees of freedom of collision partners. The specialty of the EDWSP model is that due to energy dependence in nucleus–nucleus potential it becomes more attractive across sub-barrier energies and hence predicts larger fusion excitation functions with reference to the one-dimensional barrier penetration model. The energy dependence in the nucleus–nucleus potential is also reflected in the double folding potential wherein it originates due to the underlying effective nucleon–nucleon interactions and non-local quantum effects. The non-local quantum effects are directly linked with the exchange of nucleons between fusing systems and automatically generates a velocity dependent nuclear potential [41]. Recently, the energy dependence in the nucleus–nucleus potential has also been pointed out in the microscopic time-dependent Hartree–Fock theory [42–44] wherein the nuclear potential becomes energy dependent in the domain of the Coulomb barrier. This energy dependent nucleus–nucleus potential may be a better root for understanding of the various dynamical aspects which are important for the description of near and sub-barrier fusion dynamics and hence it is reasonable to test the features of energy dependent nucleus–nucleus potential in fusion dynamics. Following this idea, the present work is an effort to address the fusion dynamics of stable nuclei, loosely (weakly) bound and halo nuclei in view of the EDWSP model.
In the fusion of reactions, the projectiles are loosely bound stable nuclei having low binding energy (BE = 1.475 MeV for
and BE = 2.45 MeV for
while the heavier projectiles (
are stable against breakup effects. The fusion dynamics of
reactions was recently measured [23, 24] wherein authors reported the suppression of above barrier fusion data with respect to coupled channel calculations (suppression factor for
reaction is 32% and suppression factor for
reaction is 24%). This suppression of above barrier fusion data can be correlated with the weakly bound nature of projectiles. To ensure this fact in addition to fusion of
reactions, the present work also analyzed the fusion dynamics of a common target with two different stable projectiles (
In the fusion of
reactions, the projectiles are stable against breakup effects. Therefore, the distinguishable features of
[23, 24] and
reactions [32–36] in above barrier region give a clear indication of projectile breakup effects. It is worth noting here that the descrpancies between theoretical calcualtions based upon static Woods–Saxon potential along with Wong formalism and experimental data can be removed if one either entertains the effects of nuclear structure degrees of freedom in coupled channel model or uses the EDWSP model along with the one-dimensional Wong formula. The coupled channel approach and the EDWSP model adequately address the sub-barrier fusion enhancement of
reactions. In the fusion of
reactions, the below barrier fusion data are reasonably explained by the EDWSP model and coupled channel model. Within the view of the EDWSP model calculations, the above barrier fusion data are suppressed by a factor of 25% for
reaction and by a factor of 17% for
reaction which is smaller than a value reported in literature [23, 24]. Furthermore, the applicability of the EDWSP model has been tested for the description of the fusion mechanism of
reaction. The
is a Borromean nucleus and the fusion dynamics of exotic
with stable
isotope has been included in the present analysis. Due to barrier modification effects, the EDWSP model adequately explains the observed fusion enhancement of this reaction and hence present calculations clearly pointed out that the coupled channel formulation and the energy dependence in nucleus–nucleus potential reflects similar behavior of heavy ion fusion reactions. The brief description of the method of calculation is given in section 2. The results are discussed in section 3 and the conclusions drawn are described in section 4.
2. Theoretical formalism
2.1. One-dimensional Wong formula
The partial wave fusion cross-section is given as

Hill and Wheeler proposed an expression for tunneling probability ( which is based upon the parabolic approximation wherein the effective interaction between the collision partners has been replaced by an inverted parabola [45]:

Using Wong's approximations in above expression and by assuming that the infinite number of partial waves contributes to the fusion process, one can obtain the following final expression for Wong formula for evaluating the fusion excitation function [39]:

2.2. Energy dependent Woods–Saxon Potential model (EDWSP model)
The effective nucleus–nucleus potential is the most sensitive input for the success of theoretical approach. In heavy ion fusion reactions, the parameterization of nuclear potential is at same footing as that of nuclear structure degrees of freedom of fusing systems. The static Woods–Saxon potential and the energy dependent Woods–Saxon potential are used along with Wong formalism for the calculations of fusion excitation functions of
and
reactions. In addition, the coupled channel calculations are performed by using static Woods–Saxon potential in order to overcome the discrepancies between theoretical predictions of one-dimensional barrier penetration model and experimental data. The form of static Woods–Saxon potential is defined as

with, The quantity 'V0' is depth and 'a' is the diffuseness parameter of nuclear potential. In the EDWSP model, the V0 is defined as [37, 38]
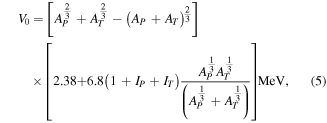
where and
are the isospin asymmetry of collision partners. In heavy ion fusion reactions, the different kinds of static and dynamical physical effects such as variations of N/Z ratio, variations of surface energy and surface diffuseness of fusing nuclei, variations of nucleon densities in the neck region and dissipation of kinetic energy of relative motion to internal structure degrees of freedom of fusing nuclei occur in the tail region of the Coulomb barrier wherein fusion start to take place. In addition, the surface region of nuclear potential (
is quite sensitive to small change of nucleon density parameters than the inner region and the nuclear structure effects of colliding nuclei are mostly present at surface region which are mainly related to the diffuseness parameter of nuclear potential. Therefore, the strengths of nuclear potential in the region near the barrier position may vary significantly due to nuclear structure effects. Such variation of nuclear potential in surface region is associated with variation of diffuseness parameter and hence in the essence of the importance of diffuseness parameter, the energy dependence in the Woods–Saxon potential is taken via its diffuseness parameter. The energy dependent diffuseness parameter is defined by the following expression
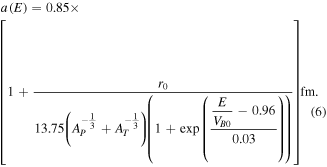
In heavy ion fusion reactions, the EDWSP model provides a wide range of diffuseness parameter depending upon the value of and bombarding energy of reactants. The range parameter (
is an adjustable parameter and its values are adjusted in order to vary the diffuseness parameter required to address of the fusion excitation function data of fusing system under consideration. It is worth noting here that the values of range parameter
strongly depend upon the nature of colliding pairs and have different value for different projectile–target combinations. In addition, the value of
also depends upon isospin asymmetry and the nature of dominant internal nuclear structure degrees of freedom of fusing nuclei.
2.3. Coupled channel model
The influence of coupling between relative motion of collision partners and nuclear structure degrees of freedom of colliding nuclei can be addressed if one incorporates channel coupling effects in coupled channel formulation [40, 46–50]. In this approach, the set of coupled channel equations are numerically solved and hence defined as
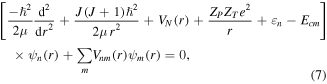
where represents the relative motion of colliding systems. μ is the reduced mass of colliding nuclei.
and
denote the bombarding energy in the centre of mass frame and the excitation energy of the nth channel, respectively. The
is the matrix elements of the coupling Hamiltonian, which in the collective model consists of Coulomb and nuclear components. In the code CCFULL [40], the coupled channel equations are numerically solved by using the no-Coriolis or rotating frame approximation and ingoing wave boundary conditions (IWBC). The no-Coriolis or rotating frame approximation is used to reduce the dimension of the coupled channel equations [40, 46–50]. By including all the dominant intrinsic channels, the fusion cross-section becomes

where is the total transmission coefficient corresponding to the angular momentum J.
3. Results and discussion
This work analyzed the fusion dynamics of stable nuclei, loosely bound and halo nuclei within the framework of the static Woods–Saxon potential and the EDWSP model by considering the fusion of
and
reactions. In addition, the coupled channel calculations are performed for entertaining the effects of intrinsic degrees of freedom of colliding nuclei. These systems are selected in such a way that the influence of the breakup channel for loosely bound projectiles (
on fusion dynamics are directly reflected. To ensure the role of projectile breakup effects, the fusion dynamics of stable projectiles (
with common target has been included in the present analysis. Furthermore, the fusion dynamics of
reaction, wherein the projectile is halo nucleus, is theoretically analyzed in the present article so that an unambiguous conclusion with regard the dominance of nuclear structure effects can be extracted. The barrier height, barrier position and barrier curvature of the fusing nuclei used in the EDWSP model calculations are listed in table 1. In table 2, the values of potential parameters such as range, depth and diffuseness used in the EDWSP model calculations for
and
reactions are listed.
Table 1.
The values of
and
used in the EDWSP model calculations for various heavy ion fusion reactions.
System |
![]() |
![]() |
![]() |
Reference |
---|---|---|---|---|
![]() |
25.51 | 9.80 | 5.04 | [23] |
![]() |
24.90 | 10.08 | 4.71 | [24] |
![]() |
61.11 | 10.84 | 4.23 | [35, 36] |
![]() |
60.76 | 10.91 | 4.10 | [35, 36] |
Table 2. Range, depth and diffuseness of Woods–Saxon potential used in the EDWSP model calculations for various heavy ion fusion reactions [37, 38].
System |
![]() |
![]() |
![]() |
---|---|---|---|
![]() |
1.040 | 32.63 |
![]() |
![]() |
1.040 | 40.51 |
![]() |
![]() |
1.110 | 67.68 |
![]() |
![]() |
1.110 | 73.76 |
![]() |
The common target isotope ( contributes equally in the fusion dynamics of
and
reactions. Therefore, the distinguish features of low energy fusion excitation functions can be understood in terms of different nuclear structure of projectiles. The inclusion of nuclear structure degrees of freedom of fusing systems leads to the substantially large sub-barrier fusion enhancement with reference to the expectations of one-dimensional barrier penetration model. The details of coupled channel calculations of
and
reactions will be discussed in figures 3 and 4. The fusion dynamics of these systems obtained by using static Woods–Saxon potential and the EDWSP model along with Wong formula are shown in figure 1. The theoretical calculations based upon static Woods–Saxon potential of
and
reactions are substantially smaller than the experimental data in below barrier energy regions. However, the predictions of the EDWSP model along with Wong formula reasonably addresses the observed fusion enhancement of these reactions in whole range of energy as depicted in figure 1. In EDWSP model, the energy dependent diffuseness parameter generates different effective potential at different bombarding energies which in turn leads to a spectrum of energy dependent effective potential barriers as shown in figure 2. In this spectrum of barriers, some barriers are lower than the height of the Coulomb barrier and consequently results in the larger enhancement of fusion excitation function at below barrier energies. Furthermore, the diffuseness parameter is also related with barrier width as well as the channel coupling strengths for the inelastic surface excitations, rotational states and nucleon transfer channels. Therefore, the variation of diffuseness parameter of Woods–Saxon potential also affects barrier width and channel coupling strengths. As far as the channel coupling strengths are concerned, these strongly depend upon the first-order derivative of static Woods–Saxon potential
[46–50].
Figure 1. The fusion excitation functions of and
reactions obtained using static Woods–Saxon potential model and the EDWSP model [37, 38]. The results are compared with available experimental data taken from [23, 24, 35, 36].
Download figure:
Standard image High-resolution imageFigure 2. The fusion barrier (FB) for (figure 2(a)) and
reactions (figures 2(b) and (c)) obtained by using EDWSP model. The similar results are found for other fusing systems.
Download figure:
Standard image High-resolution imageIn addition, as diffuseness of Woods–Saxon potential increases, the potential pocket becomes shallower and at significantly larger diffuseness parameter ( which depends upon the nature of colliding system), the potential pocket almost disappear which limits the occurrence of fusion process. In contrast to this, in EDWSP model calculations, although the potential becomes shallower with increase of diffuseness, a well-defined potential pocket still exists even at large value of diffuseness parameter (
as depicted in figure 2. The realistic coupled channel calculations (CCFULL calculations) make the use of IWBC which are quite sensitive to the existence of the potential pocket and ultimately require a deeper potential for description of the heavy ion fusion reactions. In the EDWSP model calculations, the energy dependence in diffuseness parameter, which takes care of all kinds of static and dynamical physical effects, makes it an efficient theoretical tool to address the observed fusion dynamics of various heavy ion fusion reactions.
In the fusion of systems, the EDWSP model produces largest diffuseness of
which in turn results in the lowest fusion barrier (
for
and
for
as shown in figure 2. This lowest fusion barrier is much smaller than that of corresponding Coulomb barrier as listed in table 2. For instance, in case of
system, the difference between Coulomb barrier and lowest fusion barrier produced in the EDWSP model is
(
for
Because of lowering of the fusion barrier, the incoming flux is shifted from elastic channel to fusion channel and hence prediction of anomalously larger fusion enhancement at below barrier energies is expected from the present model calculations. As the incident energy increases, the value of diffuseness parameter decreases resulting in an increase of height of the corresponding fusion barrier. In above barrier energy regions, the predictions of the different theoretical approaches overlap with each other and therefore the above barrier fusion data are almost independent of different channel coupling effects (internal structure of colliding nuclei). In view of this physical effect, in the present model calculations the value of diffuseness parameter gets saturated to its lowest value which results in highest fusion barrier as evident from figure 2. For
system, at incident energy
(well above the barrier), the highest fusion barrier produced in EDWSP model calculations is comparable with that of the Coulomb barrier while for
system, highest fusion barrier is still smaller than that of the Coulomb barrier. Therefore, the present model calculations suggest larger sub-barrier fusion enhancement of
system with respect to
system. The similar conclusions are also evident from their coupled channel analysis. The similar kinds of barrier modification effects are found for
and
reactions.
The details of coupled channel calculations of and
reactions are shown in figures 3 and 4. The deformation parameters and the corresponding excitation energies of low lying surface vibrations of target nucleus are
and
and
and
The details of coupled channel calculations of
reactions are discussed in figure 3. The inclusion of inelastic surface excitations of the target isotope (
produces dominant effect. The projectiles (
are loosely bound nuclei due to their low breakup threshold and displays the signature of the breakup channel while such effects are absent in the target isotope (
due to stability against breakup effects. The observed fusion data of
reaction are significantly larger than no coupling calculations. The addition of inelastic surface excitations of the target isotope results in a substantially larger sub-barrier fusion excitation functions over the predictions of one-dimensional barrier penetration model. The couplings to three phonon vibrational states of target isotope shift the theoretical calculations nearer to the experimental data but still there remain large deviation between sub-barrier fusion data and the coupled channel calculations. To address the below-barrier fusion data, the addition of projectile degrees of freedom must be entertained in the theoretical results. The projectile (
possess quadrupole moment
in its ground state and also the unbound first excited state
which must be incorporated in the coupled channel calculations for addressing the observed experimental data. The spectroscopic investigations indicate that the transition probability (
is equals to
for
rotational states of projectile which lies at the excitation energy of
[23–25]. In [27, 28], authors using continuum discretized coupled channel (CDCC) approach investigate the role of projectile breakup effects (
by taking projectile (
as two body
cluster structure with a breakup threshold of
This cluster structure model of projectile is found to be quite successful in CDCC calculations for description of influence of breakup channel in the fusion dynamics of
reaction. In CDCC calculations, authors [27, 28] make the use of double folding potential by viewing
reaction as
and
interactions. In nuclear surface region, the dynamic polarization potential produced due to breakup channel is of repulsive nature and consequently the authors claim the hindrance of the fusion data with respect to coupled channel calculations by a factor of 32% [23]. On the other hand, within the view of the EDWSP model calculations, the hindrance of the above barrier fusion data is minimized by a factor of 7% as inferred from figure 3(a). This suppression of above barrier fusion data can be correlated with the projectile breakup channel that arise due to its low binding energy (BE = 1.475 MeV). It is well recognized fact that the intrinsic structure degrees of freedom have negligible effects on the magnitude of above barrier fusion excitations functions data. Therefore, the overestimation of coupled channel calculations to above barrier data is the signature of the reduction of the incoming flux going into fusion channel. For
reaction, authors reported a suppression factor of 32% with respect to coupled channel calculations [23] while this suppression factor is minimized in the EDWSP model calculations wherein the above barrier fusion data are hindered by a factor of 25% which is smaller than that of 32% as depicted in figure 3(a).
Figure 3. The fusion excitation functions of reactions obtained using the EDWSP model and the coupled channel code CCFULL. The results are compared with available experimental data taken from [23, 24].
Download figure:
Standard image High-resolution imageFor reaction, the inclusion of the same vibrational degrees of freedom of target isotope as done for
system has been tested. The difference in the energy dependence of fusion cross-section of
reactions is a consequence of the different nuclear structure of projectiles. The heavier projectile (
also exhibits loosely bound characteristics but due to larger breakup threshold (BE = 2.45 MeV) in comparison to that of the lighter projectile (BE = 1.475 MeV for
the influence of breakup channel is less pronounced on the fusion dynamics of
reaction. The heavier projectile (
can be viewed as two body
cluster structure with a breakup threshold of
Therefore, the effects of breakup channel are greater for
reaction than for
reaction and consequently, the fusion hindrance at above barrier energies with reference to the coupled channel calculations is smaller for
reaction in comparison to that of
system. Within the view of CDCC calculations, the authors of [27, 28] pointed out that the effects of the breakup channel (continuum states) is to increase the height of the Coulomb barrier between the colliding nuclei and consequently results in the hindrance of the above barrier fusion data. Very recently, Rath et al [24] have performed experimental measurement of the complete fusion data of
reaction with the help of offline γ-counting technique. Theoretical analysis of the fusion data of this reaction suggests the hindrance of the above barrier fusion data by a factor of 24% with reference to the coupled channel calculations. The projectile (
exhibits quadrupole moment (Qm = −4.06 fm2) in its ground state [24] along with the unbound first excited state (
corresponding to the transition probability
is equal to
from first excited state to the ground state [24] and therefore, the inclusion of one phonon
and three phonon
vibrational states of target leads to substantially larger sub-barrier fusion excitation functions in comparison to no coupling calculations. But this coupling scheme is still unable to recover the observed fusion dynamics of
reaction. To account for the below barrier fusion data, the projectile excitations are taken into account in the coupled channel calculations. The inclusion of the projectile degrees of freedom along with inelastic surface excitations of target isotopes brings the required order of the sub-barrier fusion enhancement. However, at the cost of adequate addressal of the below barrier fusion data, the above barrier fusion data are hindered by a factor of 24% with respect to the coupled channel calculations. On the other hand, within the context of the EDWSP model calculations, the below barrier fusion data are properly addressed and the suppression of above barrier fusion data are minimized by a factor of 7%. Interestingly, the above barrier fusion data of
reaction is hindered by a factor of 17% with reference to the EDWSP model calculations, which is smaller than a value of 24% as reported in literature as inferred from the figure 3(b).
To ensure the role of projectile breakup effects, the fusion of stable projectiles ( with the common target has been discussed in figure 4. The consideration of the fusion dynamics of
systems in the present analysis is mainly for two reasons: (i) for the comparison of above barrier fusion data for weakly bound projectile and stable projectiles so that an unambiguous statement about the projectile breakup effects can extracted, (ii) for investigating the role of multi-neutron transfer channel over the inelastic surface vibrational states of reactants. In the fusion of
reactions, due to stability of projectile against breakup, the above barrier data are not suppressed with respect to theoretical predictions. At above barrier energies, the theoretical calculations due to the EDWSP model and coupled channel model adequately address the observed fusion data of
reactions as depicted in figure 4. If projectiles are taken as inert, this allows the investigation of the influence of target degrees of freedom which are the dominant mode of couplings. Therefore, for common target isotope, the distinguishable features of above barrier fusion excitation function data arises due to different characteristics of projectiles (
and
At below barrier energies, in the fusion of
reactions [35, 36], the inclusion of inelastic surface vibrations of colliding systems has a strong impact on the sub-barrier fusion enhancement. The existence of two neutron pickup channels with positive ground state Q-value for
system leads to larger sub-barrier fusion excitation function of
system with respect to
system which clearly reveals the importance of neutron transfer channel on the fusion process.
Figure 4. The fusion excitation functions of reactions obtained using the EDWSP model and the coupled channel code CCFULL. The results are compared with available experimental data taken from [35, 36].
Download figure:
Standard image High-resolution imageIn fusion of systems, no coupling calculations, wherein the colliding systems are taken as inert, are substantially smaller than that of the experimental data. For these reactions, the coupling to one phonon 2+ vibrational state or
vibrational state alone in target fails to recover the observed fusion enhancement at sub-barrier energies. The further addition of one phonon
and
vibrational states along with their mutual couplings of target isotope completely describe the sub-barrier fusion excitation function data of
system (figure 4(a)) while there remain discrepancies between theoretical calculations and experimental data for
system at sub-barrier energies (figure 4(b)). For
system (figure 4(a)), the enhancement of fusion cross-section at energies well below the Coulomb barrier is nearly four times to the expectation of single channel model which can be accurately accounted for by including the collective vibrational modes of target nucleus. However, the same coupling scheme for
system (figure 4(b)) fails to recover the below barrier fusion data. This deviation between coupled channel calculations and experimental data can be understood in terms of the possibility of two neutron pickup channel with positive ground state Q-value. Since, the target is common to both projectiles, the significant difference in the energy dependence of sub-barrier fusion cross-sections of
reactions can be correlated with the different nuclear structure of projectiles. The heavier projectile facilitates the possibility of neutron transfer channel while such characteristics are absent in the lighter projectile when they are bombarded onto a common target nucleus (
The additional sub-barrier fusion enhancement of
system with respect to
system is the direct manifestation of dominance of combined effects of inelastic surface vibrations and multi-neutron transfer channels. The similar conclusions with regard the fusion enhancement of
systems are also evident from the coupled channel analysis due to Leigh et al [35] and Morton et al [36]. It is quite interesting to note that the scenario of the fusion dynamics of
systems is adequately explained by the EDWSP model wherein the energy dependence in nucleus–nucleus potential introduces similar kinds of barrier modification effects as reflected from the coupled channel calculations.
The validity of the EDWSP model has been tested for the description of the fusion dynamics of reaction and the details of theoretical results of
reaction are shown in figure 5. In
the two valence neutrons are weakly bounded with
and hence exhibit neutron skin structure. The two neutron separation energy of
is 0.98 MeV and therefore, the wave function of its valence neutrons is largely extended in space with reference to stable nuclei but has smaller spatial extension in comparison to that of halo structure and consequently the breakup effects of
are weak in comparison to that of halo nuclei. Furthermore,
is a Borromean nucleus and the present analysis provides the theoretical description of
reaction. Kolata et al [11], performs the experimental measurement of the fusion data of
reaction and in theoretical description, the evidence of suppression of fusion data due to projectile breakup effect was pointed out. The suppression of above barrier fusion data by a factor of 6% with respect to the theoretical result was correlated with projectile breakup effects that arise due to its low breakup threshold. On the other hand, due to very weak binding energy for valence neutron of
a strong sub-barrier fusion enhancement is expected in comparison to
reactions. This sub-barrier fusion enhancement arises due to the existence of neutron transfer channels with positive ground state Q-value (Q = +2.7 MeV for one neutron transfer channel and Q = +8.8 MeV for two neutron transfer channel). Therefore, the neutron flow between colliding systems effectively lowers the fusion barrier and consequently results in the substantially larger sub-barrier fusion enhancement of
reaction over the expectations of one-dimensional barrier penetration model as depicted in figure 5. In [10], the authors pointed out that the energy dependent absorptive potential is required in order to reproduce the measured angular distribution and fusion yields. In similar fashion, the EDWSP model calculations, wherein energy dependent diffuseness parameter produces fluctuation in the effective fusion barrier, provides the complete description of the observed fusion dynamics of
reaction. Therefore, from the present analysis, one can realize that the various kinds of barrier modification effects that are induced in the presence of channel coupling effects (internal degrees of freedom of colliding pairs) are directly reflected from the EDWSP model calculations.
Figure 5. The fusion excitation functions of reaction obtained using static Woods–Saxon potential model and the EDWSP model [37, 38]. The results are compared with available experimental data taken from [11].
Download figure:
Standard image High-resolution imageIn the eigenchannel approximation, the coupling between elastic channel and nuclear structure degrees of freedom of fusing nuclei causes the splitting of the Coulomb barrier into a distribution of barrier of different heights. The shape of this barrier distribution is the fingerprint of the type of dominance of coupling involved in the fusion process. In view of barrier distribution, due to distribution of incoming flux into different channels and modification of the Coulomb barrier, the coupled channel calculations accurately describe the observed sub-barrier fusion enhancement of various heavy ion fusion reactions. Similarly, in the EDWSP model calculations, a spectrum of energy dependent barriers of varying heights is produced as shown in figure 2. As a consequence of lowering of fusion barrier between colliding systems, the present model adequately explains sub-barrier fusion enhancement of various projectile–target combinations.
4. Conclusions
To summarize, this work analyzed the fusion of stable nuclei, loosely bound and halo nuclei by studying the fusion dynamics of
and
reactions. In the fusion of
reactions, the sub-barrier fusion enhancement are completely described by the EDWSP model and coupled channel model while at above barrier energies, the fusion data are suppressed with respect to the EDWSP model calculations. Within the view of the EDWSP model calculations, the hindrance of above barrier fusion for
reactions is minimized by a factor of 7% with reference to a value reported in literature. The hindrance of above barrier fusion data can be understood in terms of projectile breakup effects that arise due to their loosely bound nature. The present calculations suggested that for
reaction, the above barrier fusion data are suppressed by a factor of 25% and for
reaction, the above barrier fusion data are hindered by a factor of 17% with respect to the EDWSP model calculations. In contrast to this, the fusion dynamics of
reactions are adequately addressed by the EDWSP model and coupled channel model in the whole range of energy. Therefore, the fusion enhancement at below barrier energies is the consequence of channel coupling effects while the fusion suppression at above barrier energies can be correlated with the loosely bound nature of projectiles. Furthermore, the validity of the EDWSP model is also tested for the description of the fusion dynamics of
reaction. Interestingly, the observed fusion enhancement of
reaction is completely described by the present model calculations. This clearly indicates that the energy dependence in Woods–Saxon potential leads to the barrier modification effects and as a consequence of lowering of fusion barrier between colliding nuclei, the EDWSP model successfully explores the fusion dynamics of
and
reactions. Furthermore for all projectile–target combinations, the theoretical calculations based upon the static Woods–Saxon potential along with Wong formula strongly underestimate the sub-barrier fusion data. However, such discrepancies can be overcome if one either incorporates the internal structure degrees of freedom of reacting nuclei in coupled channel calculations or entertains the EDWSP model along with the Wong formalism.
Acknowledgments
This work was supported by Dr D S Kothari Post-Doctoral Fellowship Scheme sponsored by University Grants Commission (UGC), New Delhi, India.