Abstract
A magnetic field is predicted to emerge on a particle in a rotating material body even if the body is electrically neutral. This emergent field is called a Barnett field. We show that nuclear magnetic resonance (NMR) enables direct measurement of the Barnett field in solids. We rotated both a sample and an NMR coil synchronously at high speed and found an NMR shift whose sign reflects that of the nuclear magnetic moments. This result provides direct evidence of the Barnett field. The use of NMR for Barnett field measurement enables the unknown signs of nuclear magnetic moments in solids to be determined.
Export citation and abstract BibTeX RIS
There are two physical mechanisms in which magnetism and mechanical rotation are combined: the Einstein–de Haas (EdH) effect1,2) and the Barnett effect.3,4) The former refers to the phenomenon in which, when a material body is magnetized by applying a magnetic field, it starts rotating as a consequence of conservation of the angular momentum of the magnetization and the body. The EdH effect has shown promise in application to nanomechanics and spintronics.5–8) The reciprocal of the EdH effect, the Barnett effect, posits that an electrically neutral body is magnetized when it is rotated. This magnetization was observed and discussed phenomenologically by introducing an emergent magnetic field (Fig. 1).3,4) Then the microscopic mechanism of this field was rigorously formulated in terms of quantum mechanics and general relativity.9–11) According to these theories, the emergent field, which originates from the coupling between an electron spin and mechanical rotation, is the essence of the magnetization related to the Barnett effect.10,11) This emergent field is hereafter called the Barnett field.
Fig. 1. Concept of a Barnett field. Magnetic fields are conventionally generated by establishing an electric current I in wires (left panel). The Barnett field is the magnetic field generated by rotating an object at the angular velocity Ω (right panel). This field is directed along the axis of rotation and appears even in electrically neutral objects.
Download figure:
Standard image High-resolution imageThe Barnett field acting on a spin emerges by coordinate transformation from the laboratory frame to the rotating one:10–12)
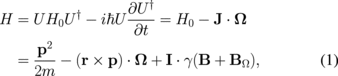
where H0 = p2/2m − I · γB is the Hamiltonian of a spin in the laboratory frame, m is the particle's mass, I is the total spin, and B is the external magnetic field. Further, γ = gq/2m is the gyromagnetic ratio, where g is the g-factor, and q is the charge. The unitary transformation is given by U = exp[iJ · Ωt/ħ], where J = r × p + I is the generator of the rotation, and Ω is the angular velocity. The last term in Eq. (1) indicates that the magnetic field B is modified by the Barnett field:

The Barnett field is proportional to the particle's rest mass. Because atomic nuclei are much more massive than electrons, relatively large Barnett fields are expected to act on nuclei. In addition, the Barnett field acting on the nucleus depends on the nuclear g-factor. Thus, a difference in the g-factor causes the magnitude of the Barnett field to vary even in the same material. In this report, we describe the direct observation of Barnett fields as a resonance shift in the nuclear magnetic resonance (NMR) under sample rotation.
The phenomenon of NMR proceeds by energy absorption and emission of an oscillating radio frequency (RF) field, Bx(ω), applied perpendicular to the direction of a static magnetic field, B0. The condition for NMR is the well-known formula

where fn, γn, and Bn are the frequency of the RF field, the nuclear gyromagnetic ratio, and the local static field acting on a nucleus, respectively.13) Neglecting small contributions from surrounding electron clouds, the local static field acting on the nucleus is the sum of an external field and the Barnett field; that is, Bn = B0 + BΩ. Thus, the value of BΩ can be measured precisely as a resonance shift by measuring either the frequency for resonance under a constant field B0 or the field for resonance under a constant frequency.14) To measure BΩ using NMR, however, the sample and the NMR detection coil should be in the same frame of reference.15) Therefore, by further extending the coil-spinning technique,16) both the sample and the tuning circuit for NMR in this experiment were rotated rapidly with respect to the direction of B0 at exactly the same angular velocity.
In Fig. 2(a), we show a schematic illustration of the experimental assembly used in this study. The assembly consists of two components: the stationary coil placed along B0 and connected to an NMR spectrometer, and a high-speed rotor consisting of a cylindrical capsule in which a specially arranged tuning circuit is installed. This circuit is composed of two small coils placed perpendicularly and connected in series, and a small capacitor. One of the two coils is arranged parallel to the stationary coil to establish coupling by mutual inductance between the tuning circuit and the stationary coil. We call this the coupling coil. The other coil, the sample coil, holds a sample inside. The RF field in the coupling coil is transmitted to the sample coil and generates the oscillating RF field Bx(ω) to induce an NMR signal. Under this configuration, the sample coil rotates at exactly the same angular velocity as the sample, thereby fulfilling the conditions for observing BΩ. The rotor is placed inside the stationary coil, and during measurements, it is rotated up to |Ω/2π| = 10 kHz. For Ω ∥ B0, the sign of Ω is defined as positive.
Fig. 2. Illustration of experimental assembly and evidence for Barnett field acting on 115In nuclei. (a) Schematic of rotor and tuning unit for measurements. Inside the rotor, the coupling coil, sample coil, and capacitor form the tuning circuit tuned at the measurement frequency. The stationary and coupling coils are coupled via mutual induction. The axes of the stationary coil, coupling coil, and rotation, and the direction of the external magnetic field are parallel to the z-axis. The axis of the sample coil is perpendicular to the z-axis. (b, c) White-scale image plots of the 115In NMR intensity obtained using the FFT-NMR method without and with rotation, respectively, at Ω/2π = +10 kHz and a measurement frequency of 27.365 MHz under various magnetic fields. White dotted line in (c) graphs the results based on Eq. (3) (see text). (d) 115In NMR intensity spectra as functions of magnetic field at measured frequencies corresponding to green line in (b) and red line in (c). (e) External magnetic field B0 dependence of the 115In NMR shifts caused by sample rotation at Ω/2π = +5 and +10 kHz. The origin of the longitudinal axis is defined as the peak position of the NMR spectrum obtained at Ω = 0.
Download figure:
Standard image High-resolution imagePowdered samples of LiF, NaCl, InP, SnO2, and Si were used for the NMR measurements. To obtain NMR signals with high S/N ratios, 119Sn and 29Si were enriched. Although 23Na and 7Li, with I = 3/2, and 115In, with I = 9/2, have quadrupole moments, no quadrupole splitting was observed in the NMR spectra because of the local cubic symmetry at the nuclear sites in these compounds. SnO2 has a tetragonal rutile crystalline structure, but 119Sn does not have a quadrupole moment. For signal acquisition, we employed a standard single π/2 pulse technique. The NMR spectra were obtained by fast Fourier transformation (FFT) of the free induction decay signals. All the measurements were performed at room temperature.
Our first step in observing the Barnett field was to record the 115In NMR shifts in the nonmetallic, nonmagnetic compound InP. To check the NMR detection using the tuning circuit described above, we measured the 115In NMR spectra as a function of the externally applied field B0 without sample rotation. The results are shown in Fig. 2(b), using a white scale for the spectrum profile. From a linear fit of the NMR center frequency to Eq. (3) with BΩ = 0, we found γn for 115In in InP to be |115γn|/2π = 9.330 MHz/T, which agrees well with the reported value of 9.3858 MHz/T.17) This fact indicates that the system was working properly to observe Barnett fields.
We then rotated the rotor while monitoring the NMR signal. In Fig. 2(c), we show the external field dependence of the NMR center frequency at an angular velocity of Ω/2π = +10 kHz parallel to the external field. The NMR center frequencies increase under rotation; that is, the nuclei feel an extra field in addition to the external field. If there is no change in 115γn with mechanical rotation, the data can be fitted to fn = |115γn(B0 + BΩ)|/2π. The Barnett field BΩ is estimated to be +1.1 ± 0.10 mT, which is represented by the orange arrows in Figs. 2(c) and 2(d). This is the first direct measurement of the Barnett field. We have confirmed that the Barnett field acting on the nuclei is independent of the external magnetic field [Fig. 2(e)], which implies that the Barnett field emerges because of rotation even under zero external magnetic field.
In Fig. 3(a), we plot the 115In NMR spectra at various values of the angular velocity Ω. The external magnetic field is fixed at B0 = 2.75 T. Clearly, the NMR center frequency increases linearly with Ω as given by Eqs. (2) and (3). Furthermore, by reversing the rotation direction (Ω < 0), the direction of the NMR shift is also reversed [compare red and blue curves in Fig. 3(a)]; thus, the sign of BΩ is reversed. The Barnett field acting on each nucleus is proportional to the angular velocity, including its sign.
Fig. 3. NMR spectra for positive and negative g-factors. Spectra for (a) 115In and (b) 29Si NMR obtained at various angular velocities. The origin of the transverse axis is defined as the peak position of the NMR spectrum obtained at Ω = 0 (orange curve). The green lines are guides to the eye.
Download figure:
Standard image High-resolution imageThe sign of the g-factor of 115In is known to be positive;17) that is, the nuclear magnetic moment is parallel to its angular momentum. Next, we measured the shifts for nuclei with negative g-factors. Equation (2) predicts that the Barnett fields reveal the sign of the g-factors. From the NMR spectra for 29Si, which has a negative g-factor,17) the direction of the NMR shift [Fig. 3(b)] is clearly opposite to that for 115In, indicating that the emergent Barnett field is opposite in direction to that for 115In. We collected the NMR shifts for various nuclei as a function of Ω (Fig. 4). In each sample, the NMR shift exhibits linear dependence on Ω. The g-factors for 7Li, 19F, 23Na, and 115In are known to be positive, whereas those for 29Si and 119Sn are negative.17) All the nuclei with positive g-factors display positive slopes (red line in Fig. 4), whereas all the nuclei with negative g-factors display a negative slope (blue line), which is consistent with Eq. (2).
Fig. 4. Universal behavior of NMR shifts as a function of the angular velocity Ω. 7Li in LiF (red dots), 19F in LiF (orange dots), 23Na in NaCl (green dots), and 115In in InP (light-blue dots) have positive g-factors. 29Si in Si (blue dots) and 119Sn in SnO2 (purple dots) have negative g-factors. Vertical axis represents NMR shifts; its origin is determined by the center frequency at Ω = 0 for each sample. Red and blue lines are theoretical predictions based on Eqs. (2) and (3) (see text).
Download figure:
Standard image High-resolution imageWe emphasize that the proposed technique realizes a new way of determining the sign of the g-factor in solids. In some nuclei, the sign is still difficult to determine.18–20) The scope of solid-state science is currently expanding to involve heavy elements. The recent discovery of NMR signals from 239Pu was highly newsworthy,18) and NMR in many nuclei remains to be discovered. For such nuclei, only minute amounts of solid are available for sampling; thus, the proposed technique may provide an exclusive method of resolving this issue because coil spinning is a unique method that enables NMR measurement of minute samples.16)
In the above discussion, we assumed that, for Eq. (2), the renormalization of the rotation effect on nuclear spins is absent in the zeroth-order approximation. With this assumption, Eq. (2) explains the experimental results within the resolution of these measurements, as demonstrated by Fig. 4. The next step is to introduce the renormalization for higher-order coupling.21) This effect modifies the nuclear g-factors; namely, the slope deviates from unity in Fig. 4. When considering these modifications, it is helpful to refer to EdH studies of electronic systems.22–24) In electronic systems, the spectroscopic g-factors are generally different from the gyroscopic g-factors. This difference arises from the fact that the gyroscopic effect combines the total angular momentum with rotation, whereas the Zeeman effect combines the total magnetic moment with a magnetic field.14) This difference enables estimation of the orbital angular momentum of electrons in solids. In contrast, modification of the nuclear g-factors reveals richer physics. The nucleus contains protons, neutrons, and mesons. All of them contribute to the orbital angular momentum, but only some can contribute to the orbital magnetic moments because neutrons are electrically neutral.25–27) Therefore, the Barnett field will provide a way to investigate such features of the nuclei, although the experimental resolution is currently not sufficient to estimate this deviation. Improving the field resolution is thus certainly worthwhile, as is applying the proposed technique to a wide range of issues.
In conclusion, we developed a new coil-spinning NMR method of detecting the Barnett field. The Barnett field is observed as an NMR shift proportional to the rotation speed. The sign of the Barnett field depends on both the sign of the nuclear magnetic moment and the rotation direction against the external magnetic field. These features can be used to determine the unknown sign of the nuclear magnetic moment. Our findings provide direct evidence of the coupling between the nuclear magnetic moment and mechanical rotation, and will produce new technology in which mechanical motion manipulates the nuclear spin angular momentum as well as the electron spin angular momentum.
Acknowledgments
The authors thank A. Nakamura, S. Nagamiya, K. Imai, and T. Maruyama for valuable discussions. This work was financially supported by a Grant-in-Aid for Scientific Research C (22540354), the Sumitomo Foundation, and Fundamental Research Grants from CREST-JST "Creation of Nanosystems with Novel Functions through Process Integration."